Autophagosome maturation: An epic journey from the ER to lysosomes
Affiliations.
- 1 Department of Molecular, Cell and Cancer Biology, University of Massachusetts Medical School, Worcester, MA [email protected].
- 2 College of Life Sciences, University of Chinese Academy of Sciences, Beijing, China [email protected].
- 3 National Laboratory of Biomacromolecules, Chinese Academy of Sciences Center for Excellence in Biomacromolecules, Institute of Biophysics, Chinese Academy of Sciences, Beijing, China.
- PMID: 30578282
- PMCID: PMC6400552
- DOI: 10.1083/jcb.201810099
Macroautophagy involves the sequestration of cytoplasmic contents in a double-membrane autophagosome and their delivery to lysosomes for degradation. In multicellular organisms, nascent autophagosomes fuse with vesicles originating from endolysosomal compartments before forming degradative autolysosomes, a process known as autophagosome maturation. ATG8 family members, tethering factors, Rab GTPases, and SNARE proteins act coordinately to mediate fusion of autophagosomes with endolysosomal vesicles. The machinery mediating autophagosome maturation is under spatiotemporal control and provides regulatory nodes to integrate nutrient availability with autophagy activity. Dysfunction of autophagosome maturation is associated with various human diseases, including neurodegenerative diseases, Vici syndrome, cancer, and lysosomal storage disorders. Understanding the molecular mechanisms underlying autophagosome maturation will provide new insights into the pathogenesis and treatment of these diseases.
© 2019 Zhao and Zhang.

Publication types
- Research Support, Non-U.S. Gov't
- Autophagic Cell Death*
- Autophagosomes / metabolism*
- Autophagosomes / pathology
- Biological Transport, Active
- Endoplasmic Reticulum / metabolism*
- Endoplasmic Reticulum / pathology
- Lysosomes / metabolism*
- Lysosomes / pathology
- Neurodegenerative Diseases / metabolism*
- Neurodegenerative Diseases / pathology
Thank you for visiting nature.com. You are using a browser version with limited support for CSS. To obtain the best experience, we recommend you use a more up to date browser (or turn off compatibility mode in Internet Explorer). In the meantime, to ensure continued support, we are displaying the site without styles and JavaScript.
- View all journals
- Explore content
- About the journal
- Publish with us
- Sign up for alerts
- Review Article
- Published: 08 November 2013
The autophagosome: origins unknown, biogenesis complex
- Christopher A. Lamb 1 ,
- Tamotsu Yoshimori 2 &
- Sharon A. Tooze 1
Nature Reviews Molecular Cell Biology volume 14 , pages 759–774 ( 2013 ) Cite this article
31k Accesses
947 Citations
12 Altmetric
Metrics details
- Autophagosomes
- Macroautophagy
Autophagy is an evolutionarily conserved lysosome-mediated degradation process that involves membrane-bound organelles called autophagosomes. Macroautophagy, commonly referred to as autophagy, is induced by amino acid starvation.
Autophagosome formation is mediated by autophagy-related (ATG) proteins. There are more than 34 ATG proteins in yeast, of which almost half are conserved in mammals.
Amino acid starvation inactivates mammalian target of rapamycin complex 1 (mTORC1), which leads to the induction of autophagy and increased autophagsome formation. Both the UNC51-like kinase (ULK) complex and the autophagy-specific class III PI3K complex are activated downstream of mTORC1 inactivation.
Autophagosome formation after amino acid starvation occurs at contact sites between the endoplasmic reticulum (ER) and mitochondria. Expansion of the site occurs on omegasomes, which are platforms that are enriched in phosphatidylinositol 3-phosphate produced by the autophagy-specific PI3K complex.
Omegasomes give rise to isolation membranes (also known as phagophores), which recruit ATG proteins, including the ULK complex, the PI3K complex, WD-repeat domain phosphoinositide-interacting 2 (WIPI2), ATG12, ATG5, ATG16L1 and LC3.
Expansion of the isolation membrane is driven by vesicular traffic from several cellular compartments, including the ER–Golgi intermediate compartment (ERGIC), the Golgi and recycling endosomes. Expansion of the isolation membrane is followed by detachment from the omegasome and closure of the vesicle around the cytosolic proteins and membranes.
Healthy cells use autophagy as a general 'housekeeping' mechanism and to survive stress, including stress induced by nutrient deprivation. Autophagy is initiated at the isolation membrane (originally termed the phagophore), and the coordinated action of ATG (autophagy-related) proteins results in the expansion of this membrane to form the autophagosome. Although the biogenesis of the isolation membrane and the autophagosome is complex and incompletely understood, insight has been gained into the molecular processes involved in initiating the isolation membrane, the source from which this originates (for example, it was recently proposed that the isolation membrane forms from the mitochondria-associated endoplasmic reticulum (ER) membrane (MAM)) and the role of ATG proteins and the vesicular trafficking machinery in autophagosome formation.
This is a preview of subscription content, access via your institution
Access options
Subscribe to this journal
Receive 12 print issues and online access
176,64 € per year
only 14,72 € per issue
Buy this article
- Purchase on Springer Link
- Instant access to full article PDF
Prices may be subject to local taxes which are calculated during checkout
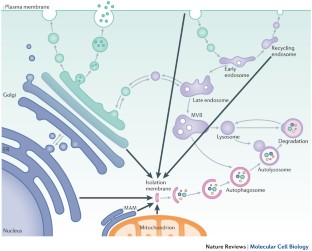
Similar content being viewed by others
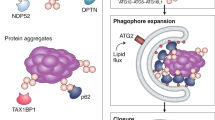
Autophagosome biogenesis comes out of the black box
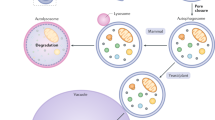
Mechanisms governing autophagosome biogenesis
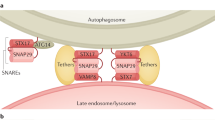
Machinery, regulation and pathophysiological implications of autophagosome maturation
Choi, A. M. K., Ryter, S. W. & Levine, B. Autophagy in human health and disease. New Engl. J. Med. 368 , 651–662 (2013).
Article CAS PubMed Google Scholar
Kuma, A. & Mizushima, N. Physiological role of autophagy as an intracellular recycling system: with an emphasis on nutrient metabolism. Seminars Cell Dev. Biol. 21 , 683–690 (2010).
Article CAS Google Scholar
Geng, J., Nair, U., Yasumura-Yorimitsu, K. & Klionsky, D. J. Post-golgi Sec proteins are required for autophagy in Saccharomyces cerevisiae . Mol. Biol. Cell 21 , 2257–2269 (2010).
Article CAS PubMed PubMed Central Google Scholar
Ohashi, Y. & Munro, S. Membrane delivery to the yeast autophagosome from the Golgi–endosomal system. Mol. Biol. Cell 21 , 3998–4008 (2010).
Bodemann, B. O. et al. RalB and the exocyst mediate the cellular starvation response by direct activation of autophagosome assembly. Cell 144 , 253–267 (2011).
van der Vaart, A., Griffith, J. & Reggiori, F. Exit from the Golgi is required for the expansion of the autophagosomal phagophore in yeast Saccharomyces cerevisiae . Mol. Biol. Cell 21 , 2270–2284 (2010).
Yamamoto, A., Masaki, R. & Tashiro, Y. Characterization of the isolation membranes and the limiting membranes of autophagosomes in rat hepatocytes by lectin cytochemistry. J. Histochem. Cytochem. 38 , 573–580 (1990).
Ravikumar, B., Moreau, K., Jahreiss, L., Puri, C. & Rubinsztein, D. C. Plasma membrane contributes to the formation of pre-autophagosomal structures. Nature Cell Biol. 12 , 747–757 (2010).
Hailey, D. W. et al. Mitochondria supply membranes for autophagosome biogenesis during starvation. Cell 141 , 656–667 (2010).
Lamb, C. A., Dooley, H. C. & Tooze, S. A. Endocytosis and autophagy: shared machinery for degradation. BioEssays 35 , 34–45 (2013).
Eskelinen, E.-L., Reggiori, F., Baba, M., Kovács, A. L. & Seglen, P. O. Seeing is believing: the impact of electron microscopy on autophagy research. Autophagy 7 , 935–956 (2011).
Novikoff, A. B. & Essner, E. Cytolysomes and mitochondrial degeneration. J. Cell Biol. 15 , 140–146 (1962).
Seglen, P. O. in Lysosomes, Their role in Protein breakdown ( eds Glaumann, H. & Ballard, F. ) 369–414 (Academic Press, 1987).
Reunanen, H., Punnonen, E. L. & Hirsimaki, P. Studies on vinblastine-induced autophagocytosis in mouse liver. V. A cytochemical study on the origin of membranes. Histochemistry 83 , 513–517 (1985).
Rez, G. & Meldolesi, J. Freeze-fracture of drug-induced autophagocytosis in the mouse exocrine pancreas. Lab. Invest. 43 , 269–277 (1980).
CAS PubMed Google Scholar
Ashford, T. P. & Porter, K. R. Cytoplasmic components in hepatic cell lysosomes. J. Cell Biol. 12 , 198–202 (1962).
Takeshige, K., Baba, M., Tsuboi, S., Noda, T. & Ohsumi, Y. Autophagy in yeast demonstrated with proteinase-deficient mutants and conditions for its induction. J. Cell Biol. 119 , 301–311 (1992).
Thumm, M. et al. Isolation of autophagocytosis mutants of Saccharomyces cerevisiae . FEBS Lett. 349 , 275–280 (1994).
Tsukada, M. & Ohsumi, Y. Isolation and characterization of autophagy-defective mutants of Saccharomyces cerevisiae . FEBS Lett. 333 , 169–174 (1993).
Liang, X. H. et al. Induction of autophagy and inhibition of tumorgenesis by beclin 1. Nature 402 , 672–676 (1999). Identifies the first link between an autophagy gene and a disease, and also the tumour-suppressive properties of autophagy.
Kuma, A. et al. The role of autophagy during the early neonatal starvation period. Nature 432 , 1032–1036 (2004). Shows that autophagy proteins are essential for surviving the neonatal starvation period in mammals and are thus essential for life.
Mizushima, N., Yoshimori, T. & Ohsumi, Y. The role of Atg proteins in autophagosome formation. Annu. Rev. Cell Dev. Biol. 27 , 107–132 (2011).
Alers, S., Löffler, A. S., Wesselborg, S. & Stork, B. Role of AMPK–mTOR–Ulk1/2 in the regulation of autophagy: cross talk, shortcuts, and feedbacks. Mol. Cell. Biol. 32 , 2–11 (2012).
McAlpine, F., Williamson, L., Tooze, S. A. & Chan, E. Y. W. Regulation of nutrient-sensitive autophagy by uncoordinated-51 like kinases 1 and 2. Autophagy 9 , 361–373 (2013).
Wirth, M., Joachim, J. & Tooze, S. A. Autophagosome formation — the role of ULK1 and Beclin1–PI3KC3 complexes in setting the stage. Seminars Cancer Biol. 23 , 301–309 (2013).
Chan, E. Y., Longatti, A., McKnight, N. C. & Tooze, S. A. Kinase-inactivated ULK proteins inhibit autophagy via their conserved C-terminal domain using an Atg13-independent mechanism. Mol. Cell. Biol. 29 , 157–171 (2009).
Ragusa, M. J., Stanley, R. E. & Hurley, J. H. Architecture of the Atg17 complex as a scaffold for autophagosome biogenesis. Cell 151 , 1501–1512 (2012).
Di Bartolomeo, S. et al. The dynamic interaction of AMBRA1 with the dynein motor complex regulates mammalian autophagy. J. Cell Biol. 191 , 155–168 (2010).
Strappazzon, F. et al. Mitochondrial BCL-2 inhibits AMBRA1-induced autophagy. EMBO J. 30 , 1195–1208 (2011).
Nazio, F. et al. mTOR inhibits autophagy by controlling ULK1 ubiquitylation, self-association and function through AMBRA1 and TRAF6. Nature Cell Biol. 15 , 406–416 (2013).
Russell, R. C. et al. ULK1 induces autophagy by phosphorylating Beclin-1 and activating VPS34 lipid kinase. Nature Cell Biol. 15 , 741–750 (2013).
Simonsen, A. & Tooze, S. A. Coordination of membrane events during autophagy by multiple class III PI3-kinase complexes. J. Cell Biol. 186 , 773–782 (2009).
Lu, Q. et al. The WD40 repeat PtdIns(3)P-binding protein EPG-6 regulates progression of omegasomes to autophagosomes. Dev. Cell 21 , 343–357 (2011).
Mauthe, M. et al. Resveratrol-mediated autophagy requires WIPI-1-regulated LC3 lipidation in the absence of induced phagophore formation. Autophagy 7 , 1448–1461 (2011).
Polson, H. E. J. et al. Mammalian Atg18 (WIPI2) localizes to omegasome-anchored phagophores and positively regulates LC3 lipidation. Autophagy 6 , 506–522 (2010).
Velikkakath, A. K. G., Nishimura, T., Oita, E., Ishihara, N. & Mizushima, N. Mammalian Atg2 proteins are essential for autophagosome formation and important for regulation of size and distribution of lipid droplets. Mol. Biol. Cell 23 , 896–909 (2012).
Baskaran, S., Ragusa, M. J., Boura, E. & Hurley, J. H. Two-site recognition of phosphatidylinositol 3-phosphate by PROPPINs in autophagy. Mol. Cell 47 , 339–348 (2012).
Krick, R. et al. Structural and functional characterization of the two phosphoinositide binding sites of PROPPINs, a β-propeller protein family. Proc. Natl Acad. Sci. USA 109 , E2042–E2049 (2012).
Article PubMed PubMed Central Google Scholar
Watanabe, Y. et al. Structure-based analyses reveal distinct binding sites for Atg2 and phosphoinositides in Atg18. J. Biol. Chem. 287 , 31681–31690 (2012).
Vergne, I. et al. Control of autophagy initiation by phosphoinositide 3-phosphatase Jumpy. EMBO J. 28 , 2244–2258 (2009).
Taguchi-Atarashi, N. et al. Modulation of local PtdIns3P levels by the PI phosphatase MTMR3 regulates constitutive autophagy. Traffic 11 , 468–478 (2010).
Klionsky, D. J. The molecular machinery of autophagy: unanswered questions. J. Cell Sci. 118 , 7–18 (2005).
Fujita, N. et al. An Atg4B mutant hampers the lipidation of LC3 paralogues and causes defects in autophagosome closure. Mol. Biol. Cell 19 , 4651–4659 (2008).
Weidberg, H. et al. LC3 and GATE-16 N termini mediate membrane fusion processes required for autophagosome biogenesis. Dev. Cell 20 , 444–454 (2011).
Orsi, A. et al. Dynamic and transient interactions of Atg9 with autophagosomes, but not membrane integration, is required for autophagy. Mol. Biol. Cell 23 , 1860–1873 (2012).
Mari, M. et al. An Atg9-containing compartment that functions in the early steps of autophagosome biogenesis. J. Cell Biol. 190 , 1005–1022 (2010).
Yamamoto, H. et al. Atg9 vesicles are an important membrane source during early steps of autophagosome formation. J. Cell Biol. 198 , 219–233 (2012).
Rao, Y. & Haucke, V. Membrane shaping by the Bin/amphiphysin/Rvs (BAR) domain protein superfamily. Cell. Mol. Life Sci. 68 , 3983–3993 (2011).
Fan, W., Nassiri, A. & Zhong, Q. Autophagosome targeting and membrane curvature sensing by Barkor/Atg14(L). Proc. Natl Acad. Sci. 108 , 7769–7774 (2011).
Matsunaga, K. et al. Autophagy requires endoplasmic reticulum targeting of the PI3-kinase complex via Atg14L. J. Cell Biol. 190 , 511–521 (2010). Isolation of an ER-targeting motif within ATG14L and evidence that recruitment of the ATG14L– PI3K complex is essential for the progression of autophagy.
Jao, C. C., Ragusa, M. J., Stanley, R. E. & Hurley, J. H. A. HORMA domain in Atg13 mediates PI 3-kinase recruitment in autophagy. Proc. Natl Acad. Sci. USA 110 , 5486–5491 (2013).
Karanasios, E. et al. Dynamic association of the ULK1 complex with omegasomes during autophagy induction. J. Cell Sci. http://dx.doi.org/10.1242/jcs.132415 (2013).
Laplante, M. & Sabatini, D. M. mTOR signaling in growth control and disease. Cell 149 , 274–293 (2012).
Sancak, Y. et al. The Rag GTPases bind Raptor and mediate amino acid signaling to mTORC1. Science 320 , 1496–1501 (2008).
Bar-Peled, L., Schweitzer, L. D., Zoncu, R. & Sabatini, D. M. Ragulator is a GEF for the rag GTPases that signal amino acid levels to mTORC1. Cell 150 , 1196–1208 (2012).
Sancak, Y. et al. Ragulator-Rag complex targets mTORC1 to the lysosomal surface and is necessary for its activation by amino acids. Cell 141 , 290–303 (2010).
Zoncu, R. et al. mTORC1 senses lysosomal amino acids through an inside-out mechanism that requires the vacuolar H + -ATPase. Science 334 , 678–683 (2011).
Dibble, C. C. et al. TBC1D7 is a third subunit of the TSC1–TSC2 complex upstream of mTORC1. Mol. Cell 47 , 535–546 (2012).
Gwinn, D. M. et al. AMPK phosphorylation of raptor mediates a metabolic checkpoint. Mol. Cell 30 , 214–226 (2008).
Egan, D. F. et al. Phosphorylation of ULK1 (hATG1) by AMP-activated protein kinase connects energy sensing to mitophagy. Science 331 , 456–461 (2011).
Kim, J., Kundu, M., Viollet, B. & Guan, K. L. AMPK and mTOR regulate autophagy through direct phosphorylation of Ulk1. Nature Cell Biol. 13 , 132–141 (2011).
Inoki, K., Li, Y., Zhu, T., Wu, J. & Guan, K. L. TSC2 is phosphorylated and inhibited by Akt and suppresses mTOR signalling. Nature Cell Biol. 4 , 648–657 (2002).
Miyazaki, M., McCarthy, J. J. & Esser, K. A. Insulin like growth factor-1-induced phosphorylation and altered distribution of tuberous sclerosis complex (TSC)1/TSC2 in C2C12 myotubes. FEBS J. 277 , 2180–2191 (2010).
Appenzeller-Herzog, C. & Hall, M. N. Bidirectional crosstalk between endoplasmic reticulum stress and mTOR signaling. Trends Cell Biol. 22 , 274–282 (2012).
Yamazaki, H. et al. Activation of the Akt–NF-κB pathway by subtilase cytotoxin through the ATF6 branch of the unfolded protein response. J. Immunol. 183 , 1480–1487 (2009).
Bellot, G. et al. Hypoxia-induced autophagy is mediated through hypoxia-inducible factor induction of BNIP3 and BNIP3L via their BH3 domains. Mol. Cell. Biol. 29 , 2570–2581 (2009).
Guo, K. et al. Hypoxia induces the expression of the pro-apoptotic gene BNIP3. Cell Death Differ. 8 , 367–376 (2001).
Papandreou, I., Lim, A. L., Laderoute, K. & Denko, N. C. Hypoxia signals autophagy in tumor cells via AMPK activity, independent of HIF-1, BNIP3, and BNIP3L. Cell Death Differ. 15 , 1572–1581 (2008).
Novak, I. et al. Nix is a selective autophagy receptor for mitochondrial clearance. EMBO Rep. 11 , 45–51 (2010).
Ashrafi, G. & Schwarz, T. L. The pathways of mitophagy for quality control and clearance of mitochondria. Cell Death Differ. 20 , 31–42 (2013).
Heider, M. R. & Munson, M. Exorcising the exocyst complex. Traffic 13 , 898–907 (2012).
Jin, R. et al. Exo84 and Sec5 are competitive regulatory Sec6/8 effectors to the RalA GTPase. EMBO J. 24 , 2064–2074 (2005).
Moreau, K., Ravikumar, B., Puri, C. & Rubinsztein, D. C. Arf6 promotes autophagosome formation via effects on phosphatidylinositol 4,5-bisphosphate and phospholipase D. J. Cell Biol. 196 , 483–496 (2012).
Moreau, K., Ravikumar, B., Renna, M., Puri, C. & Rubinsztein, David, C. Autophagosome precursor maturation requires homotypic fusion. Cell 146 , 303–317 (2011).
Itakura, E. & Mizushima, N. Characterization of autophagosome formation site by a hierarchical analysis of mammalian Atg proteins. Autophagy 6 , 764–776 (2010). Identifies the order of action of the ULK, PI3K and ATG12–ATG5–ATG16L1 complexes in autophagosome induction and formation, and their localization to the omegasome on autophagy induction.
Axe, E. L. et al. Autophagosome formation from membrane compartments enriched in phosphatidylinositol 3-phosphate and dynamically connected to the endoplasmic reticulum. J. Cell Biol. 182 , 685–701 (2008). Reveals the omegasome as a PtdIns(3)P-enriched domain of the ER, together with DFCP1 as its marker.
Vaccaro, M. I., Ropolo, A., Grasso, D. & Iovanna, J. L. A novel mammalian trans -membrane protein reveals an alternative initiation pathway for autophagy. Autophagy 4 , 388–390 (2008).
Molejon, M. I., Ropolo, A., Re, A. L., Boggio, V. & Vaccaro, M. I. The VMP1–Beclin 1 interaction regulates autophagy induction. Sci. Rep. http://dx.doi.org/10.1038/srep01055 (2013).
Hayashi-Nishino, M. et al. A subdomain of the endoplasmic reticulum forms a cradle for autophagosome formation. Nature Cell Biol. 11 , 1433–1437 (2009).
Article PubMed Google Scholar
Yla-Anttila, P., Vihinen, H., Jokitalo, E. & Eskelinen, E. L. 3D tomography reveals connections between the phagophore and endoplasmic reticulum. Autophagy 5 , 1180–1185 (2009). Shows, together with reference 79, a physical connection between the ER and the isolation membrane. Reference 79 also shows that the ER acts as a support structure for the growing organelle.
Hamasaki, M. et al. Autophagosomes form at ER–mitochondria contact sites. Nature 495 , 389–393 (2013). Implicates the ER–mitochondria contact site and the SNARE protein STX17 in autophagosome formation, through direction of the class III PI3K complex to the contact site.
Pattingre, S. et al. Bcl-2 antiapoptotic proteins inhibit Beclin 1-dependent autophagy. Cell 122 , 927–939 (2005).
Rowland, A. A. & Voeltz, G. K. Endoplasmic reticulum–mitochondria contacts: function of the junction. Nature Rev. Mol. Cell Biol. 13 , 607–625 (2012).
Itakura, E., Kishi-Itakura, C. & Mizushima, N. The hairpin-type tail-anchored SNARE syntaxin 17 targets to autophagosomes for fusion with endosomes/lysosomes. Cell 151 , 1256–1269 (2012).
van Meer, G., Voelker, D. R. & Feigenson, G. W. Membrane lipids: where they are and how they behave. Nature Rev. Mol. Cell Biol. 9 , 112–124 (2008).
Zoppino, F. C., Militello, R. D., Slavin, I., Alvarez, C. & Colombo, M. I. Autophagosome formation depends on the small GTPase Rab1 and functional ER exit sites. Traffic 11 , 1246–1261 (2010).
Ge, L., Melville, D., Zhang, M. & Schekman, R. The ER–Golgi intermediate compartment is a key membrane source for the LC3 lipidation step of autophagosome biogenesis. Elife 2 , e00947 (2013).
Graef, M., Friedman, J. R., Graham, C., Babu, M. & Nunnari, J. ER exit sites are physical and functional core autophagosome biogenesis components. Mol. Biol. Cell 24 , 2918–2931 (2013).
Suzuki, K., Akioka, M., Kondo-Kakuta, C., Yamamoto, H. & Ohsumi, Y. Fine mapping of autophagy-related proteins during autophagosome formation in Saccharomyces cerevisiae . J. Cell Sci. 126 , 2534–2544 (2013).
Webber, J. L. & Tooze, S. A. Coordinated regulation of autophagy by p38α MAPK through mAtg9 and p38IP. EMBO J. 29 , 27–40 (2010).
Young, A. R. J. et al. Starvation and ULK1-dependent cycling of mammalian Atg9 between the TGN and endosomes. J. Cell Sci. 119 , 3888–3900 (2006).
Longatti, A. et al. TBC1D14 regulates autophagosome formation via Rab11 and recycling endosomes. J. Cell Biol. 197 , 659–675 (2012).
Takahashi, Y. et al. Bif-1 regulates Atg9 trafficking by mediating the fission of Golgi membranes during autophagy. Autophagy 7 , 61–73 (2011).
Kakuta, S. et al. Atg9 vesicles recruit vesicle-tethering proteins Trs85 and Ypt1 to the autophagosome formation site. J. Biol. Chem. 287 , 44261–44269 (2012).
Mochizuki, Y. et al. Phosphatidylinositol 3-phosphatase myotubularin-related protein 6 (MTMR6) is regulated by small GTPase Rab1B in the early secretory and autophagic pathways. J. Biol. Chem. 288 , 1009–1021 (2013).
Winslow, A. R. et al. α-synuclein impairs macroautophagy: implications for Parkinson's disease. J. Cell Biol. 190 , 1023–1037 (2010).
Huang, J. et al. Antibacterial autophagy occurs at PI(3)P-enriched domains of the endoplasmic reticulum and requires Rab1 GTPase. Autophagy 7 , 17–26 (2011).
Lynch-Day, M. A. et al. Trs85 directs a Ypt1 GEF, TRAPPIII, to the phagophore to promote autophagy. Proc. Natl Acad. Sci. USA 107 , 7811–7816 (2010).
Behrends, C., Sowa, M. E., Gygi, S. P. & Harper, J. W. Network organization of the human autophagy system. Nature 466 , 68–76 (2010).
Itoh, T. et al. Golgi-resident small GTPase Rab33B interacts with Atg16L and modulates autophagosome formation. Mol. Biol. Cell 19 , 2916–2925 (2008).
Nottingham, R. M., Ganley, I. G., Barr, F. A., Lambright, D. G. & Pfeffer, S. R. RUTBC1 protein, a Rab9A effector that activates GTP hydrolysis by Rab32 and Rab33B proteins. J. Biol. Chem. 286 , 33213–33222 (2011).
Itoh, T., Kanno, E., Uemura, T., Waguri, S. & Fukuda, M. OATL1, a novel autophagosome-resident Rab33B–GAP, regulates autophagosomal maturation. J. Cell Biol. 192 , 839–853 (2011).
Kihara, A., Kabeya, Y., Ohsumi, Y. & Yoshimori, T. Beclin–phosphatidylinositol 3-kinase complex functions at the trans -Golgi network. EMBO Rep. 2 , 330–335 (2001).
Shoji-Kawata, S. et al. Identification of a candidate therapeutic autophagy-inducing peptide. Nature 494 , 201–206 (2013).
Eberle, H. B. et al. Identification and characterization of a novel human plant pathogenesis-related protein that localizes to lipid-enriched microdomains in the Golgi complex. J. Cell Sci. 115 , 827–838 (2002).
Rink, J., Ghigo, E., Kalaidzidis, Y. & Zerial, M. Rab conversion as a mechanism of progression from early to late endosomes. Cell 122 , 735–749 (2005).
Dall'Armi, C. et al. The phospholipase D1 pathway modulates macroautophagy. Nature Commun. 1 , 142 (2010).
Knævelsrud, H. et al. Membrane remodeling by the PX-BAR protein SNX18 promotes autophagosome formation. J. Cell Biol. 202 , 331–349 (2013).
Puri, C., Renna, M., Bento, C. F., Moreau, K. & Rubinsztein, D. C. Diverse autophagosome membrane sources coalesce in recycling endosomes. Cell 154 , 1285–1299 (2013).
Baxt, L. A., Garza-Mayers, A. C. & Goldberg, M. B. Bacterial subversion of host innate immune pathways. Science 340 , 697–701 (2013).
Kim, H., Lee, S. & Jung, J. When autophagy meets viruses: a double-edged sword with functions in defense and offense. Seminars Immunopathol. 32 , 323–341 (2010).
Article Google Scholar
Lamark, T. & Johansen, T. Aggrephagy: selective disposal of protein aggregates by macroautophagy. Int. J. Cell Biol. 2012 , 736905 (2012).
Iwata, J.-i. et al. Excess peroxisomes are degraded by autophagic machinery in mammals. J. Biol. Chem. 281 , 4035–4041 (2006).
Narendra, D., Tanaka, A., Suen, D.-F. & Youle, R. J. Parkin is recruited selectively to impaired mitochondria and promotes their autophagy. J. Cell Biol. 183 , 795–803 (2008).
Orenstein, S. J. & Cuervo, A. M. Chaperone-mediated autophagy: molecular mechanisms and physiological relevance. Seminars Cell Dev. Biol. 21 , 719–726 (2010).
Mijaljica, D., Prescott, M. & Devenish, R. J. Microautophagy in mammalian cells: revisiting a 40-year-old conundrum. Autophagy 7 , 673–682 (2011).
Kageyama, S. et al. The LC3 recruitment mechanism is separate from Atg9L1-dependent membrane formation in the autophagic response against Salmonella . Mol. Biol. Cell 22 , 2290–2300 (2011).
Birgisdottir, Å. B., Lamark, T. & Johansen, T. The LIR motif — crucial for selective autophagy. J. Cell Sci. 126 , 3237–3247 (2013).
Pankiv, S. et al. p62/SQSTM1 binds directly to Atg8/LC3 to facilitate degradation of ubiquitinated protein aggregates by autophagy. J. Biol. Chem. 282 , 24131–24145 (2007).
Ichimura, Y. et al. Structural basis for sorting mechanism of p62 in selective autophagy. J. Biol. Chem. 283 , 22847–22857 (2008).
Matsumoto, G., Wada, K., Okuno, M., Kurosawa, M. & Nukina, N. Serine 403 phosphorylation of p62/SQSTM1 regulates selective autophagic clearance of ubiquitinated proteins. Mol. Cell 44 , 279–289 (2011).
Filimonenko, M. et al. The selective macroautophagic degradation of aggregated proteins requires the PI3P-binding protein Alfy. Mol. Cell 38 , 265–279 (2010).
Chan, E. Y., Kir, S. & Tooze, S. A. siRNA screening of the kinome identifies ULK1 as a multidomain modulator of autophagy. J. Biol. Chem. 282 , 25464–25474 (2007).
Hara, T. et al. FIP200, a ULK-interacting protein, is required for autophagosome formation in mammalian cells. J. Cell Biol. 181 , 497–510 (2008).
Hosokawa, N. et al. Atg101, a novel mammalian autophagy protein interacting with Atg13. Autophagy 5 , 973–979 (2009).
Mercer, C. A., Kaliappan, A. & Dennis, P. B. A novel, human Atg13 binding protein, Atg101, interacts with ULK1 and is essential for macroautophagy. Autophagy 5 , 649–662 (2009).
Volinia, S. et al. A human phosphatidylinositol 3-kinase complex related to the yeast Vps34p–Vps15p protein sorting system. EMBO J. 14 , 3339–3348 (1995).
Sun, Q. et al. Identification of Barkor as a mammalian autophagy-specific factor for Beclin 1 and class III phosphatidylinositol 3-kinase. Proc. Natl Acad. Sci. USA 105 , 19211–19216 (2008).
Matsunaga, K. et al. Two Beclin 1-binding proteins, Atg14L and Rubicon, reciprocally regulate autophagy at different stages. Nature Cell Biol. 11 , U385–U369 (2009).
Tanida, I., Tanida-Miyake, E., Komatsu, M., Ueno, T. & Kominami, E. Human Apg3p/Aut1p homologue is an authentic E2 enzyme for multiple substrates, GATE-16, GABARAP, and MAP-LC3, and facilitates the conjugation of hApg12p to hApg5p. J. Biol. Chem. 277 , 13739–13744 (2002).
Kabeya, Y. et al. LC3, GABARAP and GATE16 localize to autophagosomal membrane depending on form-II formation. J. Cell Sci. 117 , 2805–2812 (2004).
Mizushima, N., Sugita, H., Yoshimori, T. & Ohsumi, Y. A new protein conjugation system in human. The counterpart of the yeast Apg12p conjugation system essential for autophagy. J. Biol. Chem. 273 , 33889–33892 (1998).
Komatsu, M. et al. Impairment of starvation-induced and constitutive autophagy in Atg7-deficient mice. J. Cell Biol. 169 , 425–434 (2005).
Kabeya, Y. et al. LC3, a mammalian homologue of yeast Apg8p, is localized in autophagosome membranes after processing. EMBO J. 19 , 5720–5728 (2000).
Mizushima, N. et al. Mouse Apg16L, a novel WD-repeat protein, targets to the autophagic isolation membrane with the Apg12–Apg5 conjugate. J. Cell Sci. 116 , 1679–1688 (2003).
Chaineau, M., Danglot, L., Proux-Gillardeaux, V. & Galli, T. Role of HRB in clathrin-dependent endocytosis. J. Biol. Chem. 283 , 34365–34373 (2008).
Sorkin, A. Cargo recognition during clathrin-mediated endocytosis: a team effort. Curr. Opin. Cell Biol. 16 , 392–399 (2004).
Brodsky, F. M., Chen, C. Y., Knuehl, C., Towler, M. C. & Wakeham, D. E. Biological basket weaving: formation and function of clathrin-coated vesicles. Annu. Rev. Cell Dev. Biol. 17 , 517–568 (2001).
Laplante, M. & Sabatini, D. M. mTOR signaling. Cold Spring Harb. Perspect. Biol. 4 , a011593 (2012).
Hara, K. et al. Raptor, a binding partner of target of rapamycin (TOR), mediates TOR action. Cell 110 , 177–189 (2002).
Divecha, N. Lipid kinases: charging PtdIns(4,5)P2 synthesis. Curr. Biol. 20 , R154–R157 (2010).
Roth, M. G. Molecular mechanisms of PLD function in membrane traffic. Traffic 9 , 1233–1239 (2008).
Hansen, C. G. & Nichols, B. J. Molecular mechanisms of clathrin-independent endocytosis. J. Cell Sci. 122 , 1713–1721 (2009).
Donaldson, J. G. Multiple roles for Arf6: sorting, structuring, and signaling at the plasma membrane. J. Biol. Chem. 278 , 41573–41576 (2003).
Praefcke, G. J. & McMahon, H. T. The dynamin superfamily: universal membrane tubulation and fission molecules? Nature Rev. Mol. Cell Biol. 5 , 133–147 (2004).
Stenmark, H. Rab GTPases as coordinators of vesicle traffic. Nature Rev. Mol. Cell Biol. 10 , 513–525 (2009).
Duran, R. V. & Hall, M. N. Regulation of TOR by small GTPases. EMBO Rep. 13 , 121–128 (2012).
Jahn, R. & Scheller, R. H. SNAREs — engines for membrane fusion. Nature Rev. Mol. Cell Biol. 7 , 631–643 (2006).
Brocker, C., Engelbrecht-Vandre, S. & Ungermann, C. Multisubunit tethering complexes and their role in membrane fusion. Curr. Biol. 20 , R943–R952 (2010).
Barrowman, J., Bhandari, D., Reinisch, K. & Ferro-Novick, S. TRAPP complexes in membrane traffic: convergence through a common Rab. Nature Rev. Mol. Cell Biol. 11 , 759–763 (2010).
Sakoh-Nakatogawa, M. et al. Atg12–Atg5 conjugate enhances E2 activity of Atg3 by rearranging its catalytic site. Nature Struct. Mol. Biol. 20 , 433–439 (2013).
Download references
Acknowledgements
C.A.L. and S.A.T. are supported by Cancer Research UK. T.Y is supported by the Japanese Ministry of Education, Culture, Sports, Science, and Technology.
Author information
Authors and affiliations.
London Research Institute, Cancer Research UK, London, WC2A 3LY, UK
Christopher A. Lamb & Sharon A. Tooze
Department of Genetics, Osaka University, Graduate School of Medicine, 2-2 Yamadaoka, Suita, Osaka, Japan
Tamotsu Yoshimori
You can also search for this author in PubMed Google Scholar
Corresponding author
Correspondence to Sharon A. Tooze .
Ethics declarations
Competing interests.
The authors declare no competing financial interests.
PowerPoint slides
Powerpoint slide for fig. 1, powerpoint slide for fig. 2, powerpoint slide for fig. 3, powerpoint slide for fig. 4, powerpoint slide for table 1, powerpoint slide for table 2.
The transition metal compound osmium tetroxide (OsO 4 ) is widely used as a fixative and to stain lipids to provide contrast in transmission electron micrographs.
(BAR domains). Crescent-shaped protein domains that bind to membranes through their concave face and may sense membrane curvature by preferentially binding curved membranes.
P40/P47phox domains. Bind phosphoinositides and are found in proteins including sorting nexins.
(pleckstrin homology domains). These domains bind phosphoinositides to target PH domain-containing proteins to specific subcellular compartments.
(HOP1, REV7 and MAD2 protein domains). May recognize DNA damage-related chromatin structures, although the HORMA domain in ATG13 may be involved in phosphoinositide binding.
(GEF). Promotes exchange of bound GDP for GTP on RAB proteins, which activates RAB and enables it to bind effectors.
(GAP). A protein family that enhances the GTP hydrolysis activity of RAB GTPase proteins. GAPs contain a TBC (TRE2–BUB2–CDC16) domain, effectively switching RAB off.
(UPR). A stress response induced in response to the accumulation of misfolded proteins in the endoplasmic reticulum lumen. Broadly, this process involves stopping the translation of new proteins and increasing the production of molecular chaperones to refold the peptide chains.
(LIR). A short peptide sequence (Ψ-Xaa-Xaa-Lys/Ile; where Ψ is an aromatic residue and Xaa is any amino acid), frequently found carboxy-terminal to acidic residues, which confers the ability to bind to members of the autophagy-related 8 (ATG8) protein family such as LC3.
A silica colloid used to perform density gradient centrifugation to isolate subcellular particles of interest.
Rights and permissions
Reprints and permissions
About this article
Cite this article.
Lamb, C., Yoshimori, T. & Tooze, S. The autophagosome: origins unknown, biogenesis complex. Nat Rev Mol Cell Biol 14 , 759–774 (2013). https://doi.org/10.1038/nrm3696
Download citation
Published : 08 November 2013
Issue Date : December 2013
DOI : https://doi.org/10.1038/nrm3696
Share this article
Anyone you share the following link with will be able to read this content:
Sorry, a shareable link is not currently available for this article.
Provided by the Springer Nature SharedIt content-sharing initiative
This article is cited by
Carnosine regulation of intracellular ph homeostasis promotes lysosome-dependent tumor immunoevasion.
- Ronghui Yan
- Pinggen Zhang
Nature Immunology (2024)
Reduction of DHHC5-mediated beclin 1 S-palmitoylation underlies autophagy decline in aging
- Jianping Liu
Nature Structural & Molecular Biology (2024)
α-Synuclein reduces acetylserotonin O-methyltransferase mediated melatonin biosynthesis by microtubule-associated protein 1 light chain 3 beta-related degradation pathway
- Congcong Jia
Cellular and Molecular Life Sciences (2024)
Evaluation of the mTORC activity in the presence of Toxoplasma gondii and azathioprine in human monocyte cell line
- Sara Nemati
- Hanieh Mohammad Rahimi
- Hamed Mirjalali
BMC Microbiology (2023)
Pannexin 1 targets mitophagy to mediate renal ischemia/reperfusion injury
- Jiahao Zhang
- Zhiyong Peng
Communications Biology (2023)
Quick links
- Explore articles by subject
- Guide to authors
- Editorial policies
Sign up for the Nature Briefing newsletter — what matters in science, free to your inbox daily.


An official website of the United States government
The .gov means it’s official. Federal government websites often end in .gov or .mil. Before sharing sensitive information, make sure you’re on a federal government site.
The site is secure. The https:// ensures that you are connecting to the official website and that any information you provide is encrypted and transmitted securely.
- Publications
- Account settings
Preview improvements coming to the PMC website in October 2024. Learn More or Try it out now .
- Advanced Search
- Journal List
- J Biomed Sci

Ubiquitin-mediated regulation of autophagy
Ruey-hwa chen.
1 Academia Sinica, Institute of Biological Chemistry, Taipei, 115 Taiwan
2 Institute of Biochemical Sciences, College of Life Science, National Taiwan University, Taipei, 100 Taiwan
Yu-Hsuan Chen
Tzu-yu huang, associated data.
Not applicable
Autophagy is a major degradation pathway that utilizes lysosome hydrolases to degrade cellular constituents and is often induced under cellular stress conditions to restore cell homeostasis. Another prime degradation pathway in the cells is ubiquitin-proteasome system (UPS), in which proteins tagged by certain types of polyubiquitin chains are selectively recognized and removed by proteasome. Although the two degradation pathways are operated independently with different sets of players, recent studies have revealed reciprocal cross talks between UPS and autophagy at multiple layers. In this review, we summarize the roles of protein ubiquitination and deubiquitination in controlling the initiation, execution, and termination of bulk autophagy as well as the role of ubiquitination in signaling certain types of selective autophagy. We also highlight how dysregulation of ubiquitin-mediated autophagy pathways is associated with a number of human diseases and the potential of targeting these pathways for disease intervention.
Introduction
Ubiquitin-proteasome system (UPS) and autophagy are two major cellular degradation machineries in eukaryotes, both of which are crucial in eliminating misfolded/unfolded proteins to maintain cell and tissue homeostasis and to prevent aging-related changes and a plethora of human diseases. In general, short-lived and soluble misfolded/unfolded proteins are targeted by UPS, whereas long-lived and insoluble protein aggregates are eliminated by autophagy [ 1 , 2 ]. The cargos of autophagy are not limited to proteins and include dysfunctional or superfluous organelles. Although the two systems are operated independently, recent studies have revealed multiple layers of interconnections between UPS and autophagy. For instance, inhibition of UPS leads to a compensatory stimulation of autophagy via several mechanisms, whereas autophagy inhibition activates or impairs proteasomal flux depending on the cellular and environmental conditions [ 3 , 4 ]. In addition, components of either system can serve as the proteolytic targets of the other system [ 4 ]. In this review, we chose to focus on the role of protein ubiquitination in regulating autophagy. Other aspects of the crosstalk between UPS and autophagy have been reviewed elsewhere [ 4 , 5 ].
Overview of protein ubiquitination
Ubiquitination is a posttranslational modification involving the conjugation of the 76 amino acid ubiquitin to the lysine residue of other proteins. This modification is mediated by the sequential action of E1 ubiquitin-activating enzyme, E2 ubiquitin conjugating enzyme, and E3 ubiquitin ligase [ 6 ]. The removal of ubiquitin from the substrate is catalyzed by a class of deubiquitinating enzymes (DUBs) [ 7 ]. Ubiquitin contains seven lysine residues and one N-terminal methionine residue, each of which can be attached to another ubiquitin moiety. As a consequence, proteins can be modified by ubiquitin monomer or polymer with different length and linkage types, making ubiquitination as one of the most elaborate and versatile posttranslational modifications [ 8 – 10 ]. In the homotypic polyubiquitination, all building blocks of the chain are connected through the same lysine or methionine residue and a total of eight different chain types can be formed. To add the complexity, heterotypic chain, which contains more than one linkage types, can also be formed and can be further categorized into mixed and branched chains. Importantly, these structurally distinct ubiquitin modifications are recognized by effector proteins with linkage-specific ubiquitin-binding domains to result in diverse functional outcomes, such as degradation, signal transduction, and alteration in subcellular localization. For instance, K48- and K11-linked chains are pivotal signals for proteasomal degradation, whereas K6, K27, K33, K63, and linear chains are usually of a nondegradative fate [ 8 – 10 ]. Recent studies have further revealed the role of branched ubiquitin chain in changing the nondegradative to degradative fate or in the enhancement of degradative signal [ 10 – 13 ].
Overview of autophagy
Autophagy is a lysosome-based degradation program activated by various cellular stresses including nutrient/energy starvation, hypoxia, ER stress, hypoxia, and organelle damage. During autophagic process, double-membrane vesicles, termed autophagosomes, are formed in the cytoplasm to sequester cellular components. This is followed by fusion of autophagosome with lysosome and degradation/recycling of sequestered cellular components to generate macromolecular building blocks [ 2 , 14 , 15 ]. The prime functions of autophagy include the removal of harmful substances (such as damaged organelles, protein aggregates, and intracellular pathogens), adaptation to metabolic stresses, and renovation during differentiation and development. Dysfunction of autophagic process has been associated with numerous diseases, including infectious diseases, cancer, neurodegeneration, cardiovascular disorders, and aging [ 16 – 18 ].
In the past decade, the molecular mechanisms of autophagy have been intensively studied. Autophagy initiation is governed by the ULK1 serine/threonine kinase which forms a complex with FIP200, ATG13, and ATG101 [ 19 , 20 ]. Upon various cellular stresses, ULK1 is activated, resulting in the phosphorylation of multiple downstream factors to trigger the autophagy cascade. One effector of ULK1 is the class III PI3K complex, which contains the lipid kinase VPS34 and regulator proteins Beclin-1, VPS15, and ATG14 [ 21 ]. ULK1 promotes the activation and the recruitment of class III PI3K complex to the autophagosome formation site (phagophore), where it generates PI3P to function in autophagosome nucleation [ 22 ]. ATG9, the only transmembrane protein in the core autophagic machinery, is thought to supply membrane to autophagosome [ 23 ]. Additionally, ATG9 binds ATG2 and WIPI proteins (ATG18 in yeast, the PI3P effectors), to participate in the early stage of autophagosome biogenesis from ER [ 24 ]. Further expansion and completion of the autophagosome depends on the two ubiquitin-like conjugation systems [ 25 ]. The ATG12 conjugation system is responsible for the conjugation of ubiquitin-like protein ATG12 to ATG5, which in turn forms a complex with ATG16L1. The ATG12-ATG5-ATG16L1 complex functions as the E3 ligase for the second conjugation system, in which the ubiquitin-like LC3 subfamily proteins (ATG8 in yeast) are conjugated to the membrane-residing phosphatidylethanolamine (PE). PE modification of the LC3 family proteins are essential for the elongation and closure of autophagosome membrane. To achieve autophagic degradation, autophagosome needs to fuse with lysosome or late endosome. The fusion requires UVRAG-containing class III PI3K complex (also known as the PI3K complex II), tethering factors such as HOPS complex, SNARE proteins such as STX17 on autophagosome membrane and VAMP8 and SNAP29 on endosome/lysosome, RAB proteins such as RAB7, and the LC3 family proteins [ 26 ]. After fusion, the inner membrane of autophagosome and materials wrapped in the inner membrane are degraded and the resulting small molecules are recycled to the cytosol.
Regulation of autophagy induction by ubiquitination and deubiquitination
Induction of autophagy needs to be tightly controlled for cells to cope with various stressed conditions. Reversible ubiquitination of the core autophagy induction factors, i.e., the subunits of ULK1 and PI3K complexes, has been revealed as a common mechanism for turning on and off the autophagy process under different cellular contexts. In addition, ubiquitination participates in positive feedback regulations for timely induction of autophagy.
The role of E3 ligases
The ubiquitin ligase TRAF6, which mediates the formation of K63-linked ubiquitin chain, plays important roles in autophagy induction. TRAF6 promotes K63 ubiquitination of ULK1, thereby enhancing ULK1 stability and function [ 27 ]. Notably, recruitment of ULK1 to TRAF6 requires the cofactor AMBRA1, a subunit of class III PI3K. Since ULK1 phosphorylates and activates AMBRA1, the TRAF6-mediated ULK1 ubiquitination participates in a positive feedback mechanism to potentiate autophagy initiation. TRAF6 also catalyzes the K63 ubiquitination of Beclin-1 [ 28 ]. This ubiquitination occurs at the BH3 domain of Beclin-1 and thus blocks Beclin-1 interaction with Bcl-2 to promote autophagy in response to the activation of Toll-like receptor (TLR) 4. Under starvation, Beclin-1 K63 ubiquitination is mediated by Cul4 E3 ligase with AMBRA1 as a substrate adaptor, thereby promoting autophagy [ 29 ]. However, the role of AMBRA1 in regulating autophagy initiation is complex. Another study reported that AMBRA1 is transiently dissociated from Cul4 at an early stage of autophagy induction. The released AMBRA1 inhibits Cul5 ubiquitin ligase, thereby stabilizing mTOR inhibitor DEPTOR [ 30 ]. Since ULK1 activity is required for the dissociation of AMBRA1 from Cul4, this regulation of AMBRA1 binding partner establishes a feedback mechanism for a rapid autophagy induction. In contrast to TRAF6 and AMBRA1, the ubiquitin ligases NEDD4 and RNF216 promote Beclin-1 proteasomal degradation to inhibit autophagy by assembling K11- and K48-linked ubiquitin chains on Beclin-1, respectively [ 31 , 32 ]. Other autophagy inducing factors also undergo degradable ubiquitination. For instance, AMBRA1 K48 ubiquitination is promoted by RNF2 E3 ligase, leading to its proteasomal degradation [ 33 ]. VPS34 and ATG14 ubiquitination and degradation are mediated by Cul1 E3 ligase containing FBXL20 and Cul3 E3 ligase containing ZBTB16 in response to DNA damage and G protein coupled signaling, respectively [ 34 , 35 ]. Together, subunits of the ULK1 and VPS34 complexes are targeted by multiple ubiquitin ligases (Fig. 1 a). While K63 ubiquitination promotes autophagy induction in response to stressed conditions or accelerates autophagy initiation through feedback mechanisms, ubiquitination by K48- and K11-linked chain types impairs autophagy induction through degradation of the core autophagic proteins.

Functional roles of ubiquitin ligases in regulating autophagy. Summary of the proteins acting in the initiation ( a ), autophagosome biogenesis ( b ), and autophagosome maturation ( c ) steps of the autophagic process that are subjected to ubiquitination by various E3 ligases. The ubiquitin chain types and the effect of ubiquitination on autophagy (promotion or inhibition) are indicated
The roles of DUBs
Among the autophagy inducing factors, Beclin-1 is a popular target for ubiquitination. Similarly, Beclin-1 appears as a hub for DUB-mediated regulation (Fig. 2 ). A20, which specifically targets the K63-linked ubiquitin chain, antagonizes the function of TRAF6 on modifying Beclin-1, thereby attenuating autophagy induction in response to TLR signaling [ 28 ]. Belcin-1 K63 ubiquitination is also negatively controlled by USP14, resulting in autophagy inhibition. Importantly, USP14 is itself activated by Akt-mediated phosphorylation and this mechanism contributes to the inhibition of autophagy activity by Akt [ 36 ]. Several DUBs influence on Beclin-1 degradable ubiquitination. For instance, USP10 and USP13 reduce Beclin-1 ubiquitination to prevent its degradation. The function of USP10 and USP13 is reversed by a chemical compound, spautin-1, which inhibits autophagy by promoting Beclin-1 degradation. Interestingly, Beclin-1 positively controls the stability of USP10 and USP13, suggesting the existence of a feedback mechanism to maintain Beclin-1 level [ 37 ]. Beclin-1 stabilization is also promoted by USP19 and ataxin 3, which specifically removes K11- and K48-ubiquitin chain from Belcin-1, respectively [ 38 , 39 ]. Finally, Beclin-1 is indirectly regulated by DUB USP33, which deubiquitinates Beclin-1 partner RALB [ 40 ]. This deubiquitination event is important for the binding of RALB with the exocyst component EXO84 and Beclin-1, which in turn drives the assembly of active ULK1 and Beclin-1-VPS34 complex for autophagy initiation [ 41 ]. The ability of Belcin-1 to be targeted by multiple DUBs highlights the importance of reversible ubiquitination in regulating autophagy initiation under different cellular contexts, even though the upstream signals regulating these deubiquitination events and the specific ubiquitin ligases that counteract these DUBs remain mostly uncharacterized.

Functional roles of DUBs in regulating autophagy initiation. Summary of DUBs that regulate autophagy initiation by targeting ULK1 or Beclin-1. The ubiquitin chain types and the effect of ubiquitination on autophagy (promotion or inhibition) are indicated
Besides Beclin-1, ULK1 is regulated by DUBs (Fig. 2 ). ULK1 K63 ubiquitination is antagonized by USP1. This function of USP1, however, regulates ULK1 cellular compartmentalization by promoting ULK1 localization to the Triton X-100 soluble fraction. Depletion of USP1 or inhibition of USP1 activity by small molecular inhibitor leads to the formation of ULK1 insoluble aggregates which also contain p62 and the aggregation marker HDAC6, thereby inhibiting canonical autophagic flux but promoting lysosome-mediated degradation of p62 [ 42 ]. The degradable ULK1 ubiquitination is reversed by USP20. Under basal conditions, USP20 maintains ULK1 level to facilitate autophagy initiation. Under prolonged starvation, the binding of USP20 to ULK1 is diminished, leading to autophagy inhibition [ 43 ]. Thus, ULK1 deubiquitination could control the dynamics of autophagy process and the decision between canonical and nonconventional autophagy.
Regulation of autophagosome biogenesis by ubiquitination
A key event for autophagosome biogenesis is the recruitment of PI3P-binding proteins to phagophore, such as WIPI2. This is followed by phagophore recruitment of ATG12-ATG5-ATG16L1 complex for the lipidation of LC3 family proteins and subsequent autophagosome expansion [ 44 , 45 ]. Recent studies have revealed that both WIPI2 and ATG16L1 are subjected to ubiquitin-mediated regulation (Fig. 1 b). Ubiquitination of ATG16L1 is mediated by gigaxonin [ 46 ], a substrate adaptor of Cul3 ubiquitin ligase mutated in a neurodegenerative disease called giant axonal neuropathy [ 47 ]. Interestingly, gigaxonin-mediated ubiquitination promotes ATG16L1 degradation through both proteasomal and autophagic routes and ATG16L1 aggregates are accumulated in gigaxonin knockout neurons. As to WIPI2, the ubiquitin ligase HUWE1 is responsible for its ubiquitination and proteasomal degradation. Importantly, targeting WIPI2 to HUWE1 requires mTORC1-dependent phosphorylation on S395 of WIPI2, uncovering a link of mTORC1 to WIPI2 degradation for autophagy inhibition [ 48 ]. During mitosis, WIPI2 ubiquitination and degradation are also potentiated. This is mediated by the Cul4 family of ubiquitin ligase, whose activity is elevated in mitosis due to increased Cul4 neddylation. Importantly, the reduction of autophagy activity in mitosis through WIPI2 ubiquitination is important for the proper progression of mitotic phase, as restoration of WIPI2 during mitosis induces mitotic slippage and cell senescence [ 49 ]. Thus, WIPI2 ubiquitination is regulated by nutrient availability and cell cycle to influence on autophagy activity.
The LC3 family protein GABARAP is itself a ubiquitin-like protein. Interestingly, GABARAP can also be modified by K48-linked ubiquitin chain through the activity of centrosome-residing ubiquitin ligase Mib1 [ 50 ] (Fig. 1 b). The centriolar satellite protein PCM1, however, binds GABARAP to protect it from Mib1-mediated ubiquitination and degradation. This stabilization of GABARAP allows it trafficking along with PCM1 from centrosome reservoir to phagophore during starvation, thereby facilitating the formation of GABARAP-positive autophagosome. Thus, the centriolar satellite controls GABARAP ubiquitination and trafficking to regulate autophagosome biogenesis.
The cysteine protease ATG4 is responsible for processing the LC3 to facilitate its lipidation and for deconjugating LC3-II at the final step of autophagy [ 51 , 52 ]. The membrane-associated ubiquitin ligase RNF5 targets a specific membrane pool of ATG4B for ubiquitination and degradation (Fig. 1 b), thereby limiting LC3 processing to restrict autophagy activity in basal condition. Upon starvation or alteration in cell redox states, the binding of RNF5 to ATG4B is attenuated, which contributes to autophagy induction [ 53 ].
The emerging role of ubiquitination in autophagosome maturation
The fusion of autophagosome with lysosome is required for autophagic flux. EPG5, a RAB7A effector, is localized to late-endosome/lysosome and promotes their fusion with autophagosome by binding to LC3 [ 54 ]. USP8, a DUB localized to the endocytic compartment, binds EPG5 and removes K63-linked ubiquitin chain from EPG5 [ 55 ]. This deubiquitination event enhances EPG5 binding to LC3, thus potentiating autophagic flux to maintain the identity of embryonic stem cell. The E3 ligase responsible for EPG5 K63 ubiquitination remains undetermined. UVRAG, a subunit of class III PI3K complex specifically required for autophagosome maturation, is modified by K29/K33 non-canonical ubiquitin chain by E3 ligase SMURF1 [ 56 ] (Fig. 1 c). This ubiquitination decreases the binding of UVRAG with its inhibitor Rubicon, thereby enhancing autophagic flux. Hence, these ubiquitination events on EPG5 and UVRAG mainly affect their interaction with other proteins, rather than promoting degradation.
Ubiquitin controls autophagy termination
Autophagy is a self-limiting process. It is turned on for cell to cope with various stressed conditions. However, once the stressed situation is resolved, autophagy machinery needs to be turned off to avoid excessive degradation. Ubiquitin-mediated degradation appears to be an ideal mechanism for terminating a cellular process, such as autophagy. Indeed, several ubiquitin-based mechanisms are involved in time-dependent or feedback regulation for autophagy termination (Fig. 3 ).

Mechanisms for ubiquitin-mediated autophagy termination. The ULK1 and VPS34 complexes are stable in the induction phase of autophagy. After the execution phase of autophagy, several components of the two complexes are degraded via direct or indirect action of indicated E3 ligases, thereby contributing to autophagy termination
The WD40 protein AMBRA1 acts as a component for both class III PI3K complex and Cul4 ubiquitin ligase complex. AMBRA1 undergoes Cul4-dependent self-ubiquitination and degradation. However, at the early stage of autophagy induction, AMBRA1 is transiently dissociated from Cul4, rendering its stabilization. AMBRA1 re-associates with Cul4 at later time points to result in its downregulation. This mechanism contributes in part to autophagy termination as expression of a Cul4-binding deficient AMBRA1 mutant leads to a prolonged autophagy response [ 30 ].
Besides Cul4 ubiquitin ligase, the Cul3 ubiquitin ligase containing KLHL20 as the substrate adaptor is found to play a major role in autophagy termination. Upon autophagy induction, the Cul3-KLHL20 complex specifically targets the autophosphorylated ULK1 for ubiquitination and degradation. Additionally, KLHL20 is recruited to phagophore where it binds and ubiquitinates VPS34 and Beclin-1. Furthermore, other subunits of the ULK1 and VPS34 complexes, such as ATG13 and ATG14, are also degraded after KLHL20-mediated degradation of their partners, even though they are not direct substrates of KLHL20. Thus, KLHL20 participates in feedback regulations for promoting the degradation of multiple autophagy inducing factors after the induction of autophagy. Depletion of KLHL20 or expression of an autophosphorylation-defective ULK1 mutant leads to a prolonged autophagy response and an increased cell death under starvation [ 57 ].
The HECT family ubiquitin ligase NEDD4L and DUB USP20 also participate in autophagy termination. During prolonged starvation, NEDD4L catalyzes the K27 and K29 ubiquitination on ULK1 [ 58 ], whereas the interaction between USP20 and ULK1 is attenuated [ 43 ]. Both mechanisms lead to downregulation of ULK1 protein level. Thus, multiple E3 ligases and DUB act in concert to limit ULK1 protein abundance, thereby contributing to autophagy termination. Importantly, the ULK1 mRNA is consistently present and its translation is induced when mTOR is reactivated by the release of building blocks from the autolysosome. This mechanism allows the recovery of ULK1 protein level for the next run of autophagy induction [ 58 ].
Ubiquitin signaling in selective autophagy
Overview of selective autophagy.
Autophagy was originally considered as a nonselective bulk degradation process, but numerous studies have later reported the selective degradation of various cellular organelles or substances via autophagy mechanism, including mitochondria, ER, peroxisome, lipid droplet, ribosome, midbody, nucleus, protein aggregate, and specific pathogens [ 59 ]. In theory, selective autophagy should result in a more specific removal of damaged or harmful cellular components and thus could be more important in disease prevention than bulk autophagy. To achieve selectivity, the cargos are often linked to LC3 family proteins directly or indirectly via ubiquitin-dependent or independent mechanisms. This review focuses only on the ubiquitin-dependent selective autophagy. Different from the bulk autophagy where protein ubiquitination often plays a modulating role, protein ubiquitination in many types of selective autophagy serves as a mark for cargo recognition and a signal for process initiation. Ubiquitinated proteins generated on the surface of cargos are responsible for the recruitment of specific autophagy adaptor proteins (also known as autophagy receptors), such as p62, OPTN, NBR1, NDP52, and TAX1BP1 [ 60 , 61 ]. Since these autophagy adaptors possess both ubiquitin-binding domain and LC3-interacting region (LIR), they function as bridges to recruit LC3 to the cargos. Certain autophagy adaptor, such as NDP52, also recruits upstream autophagy initiating complex to the cargos [ 62 , 63 ]. In this way, autophagy machinery generates autophagosome to specifically engulf the cargos. Below, we discuss the role of ubiquitination in the initiation and regulation of several types of selective autophagy (Fig. 4 ).

Ubiquitin-dependent selective autophagy. Summary of the molecular mechanisms of major types of selective autophagy using protein ubiquitination as a mark of the cargo. The E3 ligases and DUB involved in generating or removing the ubiquitin chain and the autophagy adaptors used to link ubiquitinated cargos to LC3 are indicated
The best studied ubiquitin-dependent selective autophagy mechanism is mitophagy, in which the protein kinase PINK1 and E3 ligase Parkin play a key role in building the ubiquitin chains on the outer surface of damaged mitochondria. Upon mitochondria damage, PINK1 is stabilized on mitochondria membrane to recruit Parkin [ 64 – 66 ] and phosphorylates the S65 residue on both ubiquitin and the UBL domain of Parkin, which act in concert to activate Parkin on mitochondria [ 67 – 69 ]. Parkin in turn catalyzes the ubiquitination of numerous mitochondrial outer membrane proteins [ 70 , 71 ]. Recent studies indicate that these ubiquitinated proteins not only facilitate the recruitment of autophagy adaptors but also serve as PINK1 substrates to establish a feedforward mechanism for reinforcing the PINK1-Parkin pathway [ 68 , 72 ]. Quantitative proteomic study identified numerous mitochondrial proteins whose ubiquitination is dependent on Parkin [ 73 ]. Furthermore, multiple ubiquitin chain types, such as K6, K11, K48 and K63 are generated following mitochondrial depolarization [ 68 ]. It is generally believed that the identity of the substrates is less important than the density of ubiquitin chains on mitochondria to determine the onset of mitophagy [ 74 ]. Consequently, autophagy adaptors are recruited to the damaged mitochondria. CRISPR-mediated knockout analysis on HeLa cells revealed that OPTN, NDP52 and TAX1BP1 are redundantly required for mitophagy, with OPTN playing the most prominent role [ 75 ]. OPTN further recruits TBK1 to promote mitophagy through a feedback mechanism [ 76 , 77 ]. Nevertheless, other study indicated the crucial role of p62 in Parkin-dependent autophagy in mouse macrophages and embryonic fibroblasts [ 78 , 79 ]. It is unclear whether this discrepancy is owing to the difference in the relative abundance of these adaptors in different cell types.
Besides Parkin, mitophagy can be regulated by other factors that influence on the ubiquitination of mitochondrial membrane proteins. USP30, a transmembrane DUB localized on the mitochondrial outer membrane, antagonizes the function of Parkin by removal of ubiquitin chains from mitochondria [ 80 ]. Interestingly, USP30 undergoes a Parkin-dependent monoubiquitination and proteasomal degradation, thus establishing a feedforward mechanism for Parkin to promote mitophagy. Additionally, E3 ligases other than Parkin that target mitochondrial fusion and fission machineries [ 81 , 82 ] can also regulate mitophagy, as damaged mitochondria need to go through a fission process to be enclosed into the autophagosome [ 83 ].
Peroxisomes are ubiquitous organelles involving in modulation of metabolic responses and redox regulation [ 84 ]. In mammals, damaged peroxisomes are removed through ubiquitin-dependent selective autophagy pathway [ 85 ]. Consistently, an increase in ubiquitinated proteins on the surface of peroxisomes induces pexophagy. Peroxisome membrane proteins PEX5 and PMP70 are targeted for monoubiquitination under stressed conditions through the peroxisome E3 ligase PEX2 [ 86 ]. As to the autophagy adaptors, p62 and NBR1 act in a cooperated fashion to link ubiquitinated peroxisome to autophagic machinery [ 85 , 87 ].
Although bulk autophagy and selective autophagy require the fusion with lysosome for autophagic flux, damaged lysosome is itself removed by an autophagic process called lysophagy. Lysophagy utilizes a ubiquitin-dependent selective autophagy mechanism, as ubiquitinated proteins, p62, and LC3 are all found on the surface of damaged lysosomes [ 88 , 89 ]. The damaged lysosome membranes are also decorated with galectin-3 [ 89 ], which is presumably due to the exposure of the luminal proteins to the cytosol side following membrane rupture. Recent study indicates that FBXO27, a membrane localized substrate adaptor of Cul1 ubiquitin ligase, catalyzes the ubiquitination of N-glycoproteins exposed to the damaged lysosome, thereby facilitating the recruitment of autophagy adaptor p62 [ 90 ].
In addition to cellular organelles, ubiquitin-dependent selective autophagy is also exploited to eliminate intracellular pathogens such as Salmonella, Listeria, and Mycobacterium , a process called xenophagy [ 91 ]. In the host cells, these pathogens are quickly marked by ubiquitin chains on their surface. Multiple host E3 ligases are reported to ubiquitinate pathogens. For instance, Smurf1 and Parkin are involved in the ubiquitination of M. tuberculosis [ 92 , 93 ]. LRSAM1, ARIH, and HOIPI complex are responsible for Salmonella ubiquitination [ 23 , 94 , 95 ]. Of note, the ubiquitin chain types generated by these E3 ligases are different. While LRSAM1 generates K6 and K27 chains, ARIH and HOIP1 form K48 chain and M1 chain, respectively. These different ubiquitin chains are clustered to form distinct foci on bacteria surface [ 96 ]. The M1 chain specifically recruits OPTN, whereas the recruitment of p62 and NDP52 to bacteria is independent of M1 chain, demonstrating their non-redundant functions [ 97 ]. In addition to inducing xenophagy, the M1 chain on bacteria activates NF-kB pathway to promote proinflammatory cytokine secretion, thereby inhibiting bacteria proliferation [ 96 , 97 ].
Aggrephagy is induced in response to various proteotoxic conditions, such as inhibition of proteasome or chaperons and interference with productive translation, in which aggregates of ubiquitinated proteins are observed [ 98 ]. Formation of such aggregates requires p62 [ 99 ]. Recent studies indicate that p62 drives the aggregate formation via a process called liquid-liquid phase separation [ 61 , 100 ]. In addition to the ubiquitin binding domain (UBA), p62 contains a oligomerization domain (PB1). Oligomerization of p62 allows a high-avidity binding of ubiquitinated proteins via UBA domain and finally condenses the ubiquitinated proteins into larger structures. Subsequently, P62 tethers LC3 to the condensates through its LIR to facilitate a selective sequestration of ubiquitin condensates to the autophagosome. Other autophagy adaptor, such as NBR1, can also contribute to the condensation by interacting with p62 [ 101 ].
Since ubiquitinated proteins can also be targeted to undergo proteasomal degradation, one intriguing question is how to distinguish the autophagy fate from proteasome fate. Although p62-mediated condensation may be a determining factor to direct ubiquitinated proteins to the autophagy pathway, it is worth noting that p62 can also function as a direct adaptor to recruit ubiquitinated proteins to the proteasome in cytosol or nucleus [ 102 , 103 ]. Another possibility for determining the fate of ubiquitinated protein is the quality of ubiquitin chains. It is thought that K48-ubiquitinated proteins are degraded by proteasome, whereas K63 chain modified proteins are substrates of aggrephagy. However, M1, K63, and K48 chains can all trigger phase separation in vitro via binding to p62, albeit with a lower efficiency than the K48 chain [ 61 , 100 ]. Perhaps the nature of aggrephagy substrates do not have much difference from those of the proteasome substrates and, rather, the high concentration of ubiquitin chains determines the aggrephagy fate by favoring a p62-mediated phase separation [ 101 ].
Ubiquitin-mediated autophagy regulation in diseases
Neurodegenerative diseases.
There is increasing evidence for the association of autophagy dysfunction with various neurodegenerative diseases, such as Alzheimer’s disease, tauopathies, Parkinson’s disease (PD), polyglutamine disorders, and amyotrophic lateral sclerosis [ 104 ]. The most well-known neurodegenerative disease associated with defects in ubiquitin-mediated autophagy is PD, which is the second most common late-onset neurodegenerative disease resulted from the loss of dopaminergic neurons in the substantia nigra pars compacta. Mutations in genes encoding either PINK1 or Parkin are associated with autosomal recessive forms of PD [ 105 ]. Mice deficient in either Parkin or PINK1 exhibit mitochondrial impairments, but most of them cannot recapitulate the prime features of human PD, that is, loss of dopaminergic neurons [ 106 , 107 ]. A recent study generated by Parkin homozygous knockout in the background of mice with the expression of a proof-reading defective mtDNA polymerase (called mutator mice). The combination of Parkin knockout and mtDNA mutation leads to the loss of dopaminergic neurons selectively in the substantia nigra and motor defect [ 108 ]. This genetic evidence, in conjunction with the mitochondrial dysfunction found in brain and other organs of PD patients [ 106 ], point out the importance of mitophagy in PD etiology.
Another type of neurodegenerative disorder is caused by mutant proteins with the expansion of continuous stretches of glutamine (called polyQ repeats), including Huntington’s disease, spinocerebellar ataxia (SCA), and spinal and bulbar muscular atrophy [ 104 ]. A recent study uncovered a link of ubiquitin-mediated autophagy regulation to various polyQ diseases. Ataxin 3 is a polyQ-containing DUB and its polyQ expansion is associated with SCA type 3, in which neurodegeneration occurs in the striatum and cerebellum [ 109 ]. Interestingly, the normal function of ataxin 3 is to remove the polyubiquitin chain from Beclin-1, leading to its stabilization [ 38 ]. With this function, ataxin 3 is required for starvation-induced autophagy. Importantly, several proteins with expanded polyQ repeats, including ataxin 3 itself, can compete with ataxin 3 for binding Beclin-1, in a polyQ length-dependent fashion. Furthermore, although ataxin 3 with expanded polyQ repeats elicits higher binding affinity to Beclin-1, it is defective in removing ubiquitin chain from Beclin-1. Thus, these findings identify a link of ataxin 3 to autophagy regulation and, more importantly, suggest that impairment of Beclin-1-mediated autophagy accounts for one mechanism of polyQ repeat-associated neurodegenerative diseases.
Infectious diseases and inflammation
As described above, ubiquitin serves as a tag to facilitate the autophagic degradation of intracellular pathogens (xenophagy) and a number of ubiquitin E3 ligases are involved in the addition of such tag. Since autophagy core machinery is also required for the xenophagy process, regulators that affect ubiquitin-dependent turnover of autophagic core factors could also control xenophagy. For instance, RNF216, which targets Beclin-1 for ubiquitination and degradation, promotes Listeria monocytogenes proliferation and distribution in cell and mouse models [ 32 ]. Nevertheless, it should be noted that the bulk autophagy could elicit housekeeping function to restrict inflammation, thereby favoring pathogen survival [ 91 ]. The balance between selective autophagy and anti-inflammation could determine the outcome of infection and immunological functions. One example for ubiquitination-mediated balance of anti-infection arm and anti-inflammation arm lies in USP19-depedent Beclin-1 deubiquitination [ 39 ]. On one hand, this deubiquitination stabilizes Beclin-1 to favor autophagy-dependent pathogen clearance. On the other hand, the stabilized Beclin-1 binds to the CARD domain of MAVS to prevent MAVS-RIG-I association, thereby inhibiting type I interferon production and anti-viral immunity.
Liver disease, metabolic syndromes and cancer
Autophagy is important in controlling hepatocyte lipid metabolism to maintain normal liver functions [ 110 ]. Autophagy deficiency by ATG7 knockout aggravates liver steatosis induced by high fat diet and promotes the development of liver adenoma [ 111 ]. Conversely, liver steatosis impairs autophagy through ATG7 downregulation [ 112 ]. One important function of autophagy to regulate lipid metabolism is the turnover of lipid droplets via a selective autophagy process called lipophagy [ 111 ]. Similar to other selective autophagy processes, lipophagy requires certain core autophagic factors. A recent study reveals an inhibitory role of HUWE1-mediated WIPI2 degradation in lipid droplet turnover in the liver, leading to the accumulation of liver neural lipids [ 48 ]. Besides liver disease, ubiquitin-mediated autophagy regulation is implicated in other metabolic syndromes. For instance, failure of autophagy termination by KLHL20 deficiency potentiates muscle atrophy in diabetes mouse model [ 57 ].
Autophagy plays complex roles in cancer, which may depend on the different stages of cancer development. In the tumor initiating stage, autophagy suppresses carcinogenesis. However, once tumor is formed, tumor cells exploit the autophagic process for them to survive in the harsh environments [ 17 ]. The impact of ubiquitin-mediated autophagy regulation on tumor formation and progression is poorly studied. A recent study reported that the Smurf1-induced UVRAG ubiquitination promotes not only autophagosome maturation but hepatocellular carcinoma (HCC) growth [ 56 ]. Furthermore, phosphorylation of UVRAG at S522, which disrupts Smurf1 binding, correlates with poor survival of HCC patients. These findings support a tumor suppressive role of autophagy in HCC.
Conclusion and perspectives
In this review, we discussed the impact of protein ubiquitination in autophagy regulation. Protein ubiquitination serves as an ‘eat me’ signal for many types of selective autophagy by recruiting autophagic adaptors and subsequently the core autophagic proteins. In contrast to the “signaling” role of ubiquitination in selective autophagy, protein ubiquitination mainly plays modulating role in almost every step of bulk autophagy. The initiation and nucleation steps of autophagosome formation are most prevalently regulated by ubiquitination, meaning that ubiquitination controls the onset of autophagic process in response to various stressed conditions. Nevertheless, later steps of autophagosome formation and autophagosome maturation are also subjected to ubiquitin-mediated regulation. Furthermore, ubiquitin-mediated protein turnover has been used as a prime mechanism for autophagy termination under prolonged stress conditions, thereby preventing the detrimental effect of excessive autophagic degradation. The pleiotropic role of protein ubiquitination in autophagy regulation highlights the tight crosstalk between the two major cellular degradation machineries.
Dysregulation of ubiquitin-mediated autophagy process has been implicated in many disease states, such as neurodegeneration, infectious diseases, liver diseases and metabolic syndromes. With the important role of autophagy in maintaining normal physiology and homeostasis, it is expected to uncover further linkages between dysregulation of ubiquitin-mediated autophagy pathways and various human diseases, especially for age-related diseases. In this regard, targeting of these pathways by modulating the activity of E3 ligase or DUB could be exploited as a strategy for disease intervention and has been an area receiving considerable attention. For example, the small molecular inhibitor of USP10 and USP13, called spautin-1, is capable of antagonizing the ubiquitination and degradation of Beclin-1 and p53, two tumor suppressor proteins, and therefore is a promising anti-cancer agent [ 37 ]. In the future, an improved understanding of how ubiquitin-mediated autophagy regulation contributes to the pathology of human diseases and the development of less toxic and more specific agents will benefit more patients.
Acknowledgements
Abbreviations, authors’ contributions.
All authors collected and reviewed literatures and wrote the manuscript. Y-H C and T-Y H designed and illustrated figures. All authors read and approved the final manuscript.
This work was supported by MOST Frontier Grant MOST106–2321-B-001-009 and an intramural fund from Institute of Biological Chemistry, Academia Sinica.
Availability of data and materials
Ethics approval and consent to participate, consent for publication, competing interests.
The authors declare that they have no competing interests.
Publisher’s Note
Springer Nature remains neutral with regard to jurisdictional claims in published maps and institutional affiliations.
Contributor Information
Ruey-Hwa Chen, Email: wt.ude.acinis.etag@nehchr .
Yu-Hsuan Chen, Email: moc.liamg@424tsebod .
Tzu-Yu Huang, Email: moc.liamg@2120080i .
Autophagosome maturation: An epic journey from the ER to lysosomes.
Macroautophagy involves the sequestration of cytoplasmic contents in a double-membrane autophagosome and their delivery to lysosomes for degradation. In multicellular organisms, nascent autophagosomes fuse with vesicles originating from endolysosomal compartments before forming degradative autolysosomes, a process known as autophagosome maturation. ATG8 family members, tethering factors, Rab GTPases, and SNARE proteins act coordinately to mediate fusion of autophagosomes with endolysosomal vesicles. The machinery mediating autophagosome maturation is under spatiotemporal control and provides regulatory nodes to integrate nutrient availability with autophagy activity. Dysfunction of autophagosome maturation is associated with various human diseases, including neurodegenerative diseases, Vici syndrome, cancer, and lysosomal storage disorders. Understanding the molecular mechanisms underlying autophagosome maturation will provide new insights into the pathogenesis and treatment of these diseases.
View Article From Source
Similar Publications

Autophagosome maturation: An epic journey from the ER to lysosomes
Abstract: macroautophagy involves the sequestration of cytoplasmic contents in a double-membrane autophagosome and their delivery to lysosomes for degradation. in multicellular organisms, nascent autophagosomes fuse with vesicles originating from endolysosomal compartments before forming degradative autolysosomes, a process known as autophagosome maturation. atg8 family members, tethering factors, rab gtpases, and snare proteins act coordinately to mediate fusion of autophagosomes with endolysosomal vesicles. the machin… show more.
Search citation statements
Paper Sections
Citation Types
Year Published
Publication Types
Relationship
Cited by 252 publication s
References 133 publication s, curvature-sensitive trans -assembly of human atg8-family proteins in autophagy-related membrane tethering.
In macroautophagy, de novo formation of the double membrane-bound organelles, termed autophagosomes, is essential for engulfing and sequestering the cytoplasmic contents to be degraded in the lytic compartments such as vacuoles and lysosomes. Atg8-family proteins have been known to be responsible for autophagosome formation via membrane tethering and fusion events of precursor membrane structures. Nevertheless, how Atg8 proteins act directly upon autophagosome formation still remains enigmatic. Here, to further gain molecular insights into Atg8-mediated autophagic membrane dynamics, we study the two representative human Atg8 orthologs, LC3B and GATE-16, by quantitatively evaluating their intrinsic potency to physically tether lipid membranes in a chemically defined reconstitution system using purified Atg8 proteins and synthetic liposomes. Both LC3B and GATE-16 retained the capacities to trigger efficient membrane tethering at the protein-to-lipid molar ratios ranging from 1:100 to 1:5,000. These human Atg8mediated membrane tethering reactions require transassembly between the membrane-anchored forms of LC3B and GATE-16 and can be reversibly and strictly controlled by the membrane attachment and detachment cycles. Strikingly, we further uncovered distinct membrane curvature dependences of LC3B-and GATE-16-mediated membrane tethering reactions: LC3B can drive tethering more efficiently than GATE-16 for highly-curved small vesicles (e.g. 50 nm in diameter), although GATE-16 turns out to be a more potent tether than LC3B for flatter large vesicles (e.g. 200 and 400 nm in diameter). Our findings establish curvature-sensitive trans-assembly of human Atg8-family proteins in reconstituted membrane tethering, which recapitulates an essential subreaction of the biogenesis of autophagosomes in vivo.
A conformational switch driven by phosphorylation regulates Ykt6 activity in macroautophagy
1Membrane fusion, an essential process in all eukaryotes, is driven by SNARE proteins. Ykt6 is an 2 essential SNARE that plays critical roles throughout the secretory, endocytic, and autophagy 3 pathways. Ykt6 activity is thought to be regulated by a conformational change from a closed 4 cytosolic form to an open membrane-bound form, yet the mechanism that regulates this transition 5 is unknown. Through genetic, pharmacologic, and structural modeling approaches in mammalian 6 cells, we found that phosphorylation regulates Ykt6 conversion from a closed to an open state. 7The phosphorylation site we identified is highly conserved in evolution and is regulated by the 8 Ca 2+ -dependent phosphatase, calcineurin. We found that phosphorylation is a key determinant 9 for intracellular localization of Ykt6 and its function in macroautophagy. Our studies reveal a novel 10 mechanism by which Ykt6 conformation and activity is regulated by Ca 2+ signaling with 11 implications in Parkinson's Disease in which Ykt6 has been shown to play a role.
ESCRT machinery mediates selective microautophagy of endoplasmic reticulum
ER‐phagy, the selective autophagy of endoplasmic reticulum (ER), safeguards organelle homeostasis by eliminating misfolded proteins and regulating ER size. ER‐phagy can occur by macroautophagic and microautophagic mechanisms. While dedicated machinery for macro‐ER‐phagy has been discovered, the molecules and mechanisms mediating micro‐ER‐phagy remain unknown. Here, we first show that micro‐ER‐phagy in yeast involves the conversion of stacked cisternal ER into multilamellar ER whorls during microautophagic uptake into lysosomes. Second, we identify the conserved Nem1‐Spo7 phosphatase complex and the ESCRT machinery as key components for micro‐ER‐phagy. Third, we demonstrate that macro‐ and micro‐ER‐phagy are parallel pathways with distinct molecular requirements. Finally, we provide evidence that the ESCRT machinery directly functions in scission of the lysosomal membrane to complete the microautophagic uptake of ER. These findings establish a framework for a mechanistic understanding of micro‐ER‐phagy and, thus, a comprehensive appreciation of the role of autophagy in ER homeostasis.
scite is a Brooklyn-based organization that helps researchers better discover and understand research articles through Smart Citations–citations that display the context of the citation and describe whether the article provides supporting or contrasting evidence. scite is used by students and researchers from around the world and is funded in part by the National Science Foundation and the National Institute on Drug Abuse of the National Institutes of Health.
Contact Info
10624 S. Eastern Ave., Ste. A-614
Henderson, NV 89052, USA
Blog Terms and Conditions API Terms Privacy Policy Contact Cookie Preferences Do Not Sell or Share My Personal Information
Copyright © 2024 scite LLC. All rights reserved.
Made with 💙 for researchers
Part of the Research Solutions Family.
Europe PMC requires Javascript to function effectively.
Either your web browser doesn't support Javascript or it is currently turned off. In the latter case, please turn on Javascript support in your web browser and reload this page.
Search life-sciences literature (44,064,784 articles, preprints and more)
- Free full text
- Citations & impact
- Similar Articles
Autophagosome maturation: An epic journey from the ER to lysosomes.
Author information, affiliations, orcids linked to this article.
- Zhang H | 0000-0002-3342-5377
- Zhao YG | 0000-0002-4588-6752
The Journal of Cell Biology , 21 Dec 2018 , 218(3): 757-770 https://doi.org/10.1083/jcb.201810099 PMID: 30578282 PMCID: PMC6400552
Abstract
Free full text .

Autophagosome maturation: An epic journey from the ER to lysosomes
Yan g. zhao.
1 Department of Molecular, Cell and Cancer Biology, University of Massachusetts Medical School, Worcester, MA
2 College of Life Sciences, University of Chinese Academy of Sciences, Beijing, China
3 National Laboratory of Biomacromolecules, Chinese Academy of Sciences Center for Excellence in Biomacromolecules, Institute of Biophysics, Chinese Academy of Sciences, Beijing, China
Macroautophagy involves the sequestration of cytoplasmic contents in a double-membrane autophagosome and their delivery to lysosomes for degradation. In multicellular organisms, nascent autophagosomes fuse with vesicles originating from endolysosomal compartments before forming degradative autolysosomes, a process known as autophagosome maturation. ATG8 family members, tethering factors, Rab GTPases, and SNARE proteins act coordinately to mediate fusion of autophagosomes with endolysosomal vesicles. The machinery mediating autophagosome maturation is under spatiotemporal control and provides regulatory nodes to integrate nutrient availability with autophagy activity. Dysfunction of autophagosome maturation is associated with various human diseases, including neurodegenerative diseases, Vici syndrome, cancer, and lysosomal storage disorders. Understanding the molecular mechanisms underlying autophagosome maturation will provide new insights into the pathogenesis and treatment of these diseases.
Introduction
Macroautophagy (hereafter autophagy) refers to the engulfment of cytoplasmic contents, such as a portion of cytosol, mitochondria, or the ER, in a double-membrane autophagosome and their delivery to the vacuole (in yeast) or lysosomes (in multicellular organisms) for degradation. Formation of the autophagosome involves multiple membrane remodeling processes, including initiation and nucleation of a cup-shaped isolation membrane (IM; also known as the phagophore) and its subsequent expansion and closure ( Nakatogawa et al., 2009 ; Lamb et al., 2013 ; Feng et al., 2014 ). By degrading and recycling sequestrated materials, autophagy acts as a survival mechanism in response to a variety of stress conditions. Autophagy also removes damaged organelles and/or misfolded proteins in a highly selective manner to maintain cellular homeostasis. Selective autophagy requires adaptor molecules that link the cargo to the autophagic machinery ( Stolz et al., 2014 ; Anding and Baehrecke, 2017 ).
Our basic understanding of the molecular mechanism of autophagosome formation mainly comes from the study of a set of autophagy-related ( ATG ) genes identified from yeast genetic screens ( Nakatogawa et al., 2009 ; Lamb et al., 2013 ; Feng et al., 2014 ; Zhao and Zhang, 2018 ). ATG proteins form different complexes and act coordinately for autophagosome biogenesis. In brief, the ATG1 complex (composed of ATG1, ATG13, and ATG17) and the class III phosphatidylinositol 3-phosphate (PI(3)P) kinase complex (composed of VPS34, VPS15, ATG6/Beclin1, and ATG14) are required for initiation and nucleation of IMs. Vesicles carrying the integral membrane protein ATG9, known as ATG9 vesicles, may provide the membrane source for initiation of IMs. ATG9 is later retrieved from autophagosomal membranes, a process controlled by the ATG2/ATG18 complex. The two ubiquitin-like conjugation systems, including ATG7 (E1 enzyme)/ATG3 (E2 enzyme)/Atg8 family members (ubiquitin-like molecules) and ATG7/ATG10 (E2 enzyme)/ATG12 (ubiquitin-like molecule), function in autophagosomal membrane expansion and closure ( Nakatogawa et al., 2009 ; Lamb et al., 2013 ; Feng et al., 2014 ).
The autophagy pathway in multicellular organisms is much more complex, containing steps that are not present in yeast ( Lamb et al., 2013 ; Zhang and Baehrecke, 2015 ; Zhao and Zhang, 2018 ). For example, in yeast, autophagosomes are generated at a single site, called the preautophagosomal formation site, and directly fuse with the vacuole. In multicellular organisms, autophagosomes are formed simultaneously at different sites, and nascent autophagosomes fuse with vesicles originating from the endolysosomal compartments before eventually forming degradative autolysosomes. Lysosomes are regenerated after the sequestrated materials are degraded. In this review, we will discuss recent advances in understanding the mechanism underlying the conversion of nascent autophagosomes into functional autolysosomes.
It is useful to clarify a few terms describing the steps after autophagosome formation. Autophagosome maturation refers to the process involving the progression of nascent autophagosomes to degradative autolysosomes. It is often misused to refer to progression from IMs to autophagosomes. The term amphisome refers to structures containing both autophagic and endocytic materials, while the term autolysosome indicates structures containing autophagic and lysosome-specific contents such as hydrolases ( Eskelinen, 2005 ). Although LAMP1 is routinely used as a marker for lysosomes, LAMP1-labeled compartments are actually heterogeneous in nature ( Cheng et al., 2018 ). Lysosomal membrane proteins such as LAMP1 and LAMP2 are transported from the trans-Golgi network by vesicles, and they can accumulate in vesicles of different compartments if the trafficking process is impaired. Therefore, assays monitoring acidification and lysosomal hydrolases are more accurate than LAMP1/2-labeling methods to distinguish autolysosomes from autophagosomes/amphisomes. The term autophagic vacuole (AV) refers collectively to autophagic structures after autophagosome formation, including autophagosomes, amphisomes, and autolysosomes.
Autophagosomes are formed at the ER
In multicellular organisms, the ER functions as a platform for the recruitment of ATG proteins for autophagosome formation. Upon autophagy induction, the ULK1/FIP200 complex (the mammalian Atg1 complex) is targeted to the ER and recruits the PI(3)P kinase complex for generation of PI(3)P ( Itakura and Mizushima, 2010 ; Zhao and Zhang, 2018 ). The specialized PI(3)P-enriched subdomains of the ER, named omegasomes, provide platforms for IM nucleation and expansion ( Fig. 1 ; Axe et al., 2008 ). The possible membrane sources for the initial nucleation of IMs include COPII vesicles, ATG9 vesicles, and membranes originating from omegasome structures ( Lamb et al., 2013 ; Karanasios et al., 2016 ). Plasma membrane, mitochondria, ER–Golgi intermediate compartment, COPII vesicles, and ATG9 vesicles have all been suggested to contribute membrane sources for IM expansion ( Hailey et al., 2010 ; Ravikumar et al., 2010 ; Orsi et al., 2012 ; Ge et al., 2013 ; Graef et al., 2013 ; Puri et al., 2013 ; Zhao and Zhang, 2018 ). The IM is closely apposed with the ER and is also linked to the ER by tubular membrane extensions, collectively referred to as ER–IM contacts ( Hayashi-Nishino et al., 2009 ; Ylä-Anttila et al., 2009 ), at which lipid transfer between the ER and IMs may occur ( Prinz, 2014 ; Phillips and Voeltz, 2016 ; Zhang and Hu, 2016 ). Several factors have been identified that mediate the formation of dynamic ER–IM contacts. The two ER-resident tail-anchored VAP proteins (VAPA and VAPB) interact with FIP200 and ULK1 through their conserved FFAT motifs (two phenylalanines in an acidic tract), contributing to the recruitment of ULK1 and also stabilization of the ULK1/FIP200 complex at the autophagosome formation sites on the ER ( Zhao et al., 2018 ). The interaction between FIP200 and the WD40-repeat containing PI(3)P-binding protein WIPI2 (one of four mammalian ATG18 homologues) also participates in mediating the ER–IM contact ( Zhao et al., 2017 , 2018 ). Yeast ATG2 tethers the ER to the leading edges of the growing IMs by simultaneously binding to the ER and also acting cooperatively with Atg18 to bind ATG9 at the IM extremities ( Gómez-Sánchez et al., 2018 ; Kotani et al., 2018 ). Whether mammalian ATG2 also participates in ER–IM contact formation has yet to be determined. The ER-transmembrane protein EPG-3/VMP1 modulates disassembly of ER–IM contacts. VMP1 activates the ER Ca 2+ channel SERCA to disassociate IMs from the ER, probably through perturbation of local Ca 2+ concentration ( Zhao et al., 2017 ). VMP1 depletion causes stable association of IMs with the ER, and the IMs then fail to proceed into closed autophagosomes ( Zhao et al., 2017 ).

Overview of the autophagy pathway. Autophagosomes are generated at PI(3)P-enriched subdomains of the ER, called omegasomes (Ω). A cup-shaped autophagosomal precursor structure, the IM, forms in close association with the omegasome. Upon closure of the IM, cytoplasmic contents are enclosed in double-membrane autophagosomes, also known as AVi. Autophagosomes undergo a series of fusion processes with various endolysosomal compartments, including the earliest vesicular endocytic vesicles (EVE), early endosomes (EE), MVBs, and LEs/lysosomes to form amphisomes, also known as AVi/d. Amphisomes finally mature into functional autolysosomes, also called AVd.
The final closure of IMs into autophagosomes appears to be completed by membrane abscission mediated by the endosomal sorting complex required for transport (ESCRT; Yu and Melia, 2017 ; Takahashi et al., 2018 ) and is accompanied by a change in morphology from elliptical expanding IMs to spherical sealed autophagosomes ( Tsuboyama et al., 2016 ). This process is impaired in cells deficient in the ESCRT machinery and also the ATG8 conjugation system ( Tsuboyama et al., 2016 ; Takahashi et al., 2018 ). Upon closure, nascent autophagosomes dissociate from the ER and undergo maturation.
Autophagosome maturation involves multiple entry sites for endocytic vesicles
AVs can be classified into three types in EM analysis based on their morphology and enzymatic characteristics. Nascent autophagosomes, termed AV-initial (AVi), consist of double-membrane structures containing unaltered cytoplasmic constituents. AV-intermediates (AVi/ds) represent amphisomes with a single limiting membrane that shows no or early signs of content degradation, while AV-degradative (AVd) structures are autolysosomes containing components at various stages of degradation ( Eskelinen, 2005 ; Fig. 1 ). A series of elegant EM analyses using different endosome labeling methods were performed in the 1990s to determine the stage of the autophagic and endocytic pathway when the fusion occurs ( Dunn, 1990 ; Tooze et al., 1990 ; Yokota et al., 1995 ). Dunn (1990) revealed that maturation of AVs into degradative vacuoles in rat liver cells occurs in a stepwise fashion by sequential acquisition of lysosome membrane proteins and lysosomal hydrolases and acidification of the lumen. These steps appear to involve fusion with different stages of endolysosomal compartments ( Dunn, 1990 ). Tooze et al. (1990) showed that the endocytic tracer HRP (a marker of fluid phase endocytosis) is first detected in AVi/ds (amphisomes) in pig exocrine pancreatic cells, indicating the convergence of early endosomes and AVis. Using immunogold cytochemistry and morphometric analysis, Liou et al. (1997) also found that in rat liver cells, AVis coalesce with vesicular endosomes, including small vesicles of the size of the earliest endocytic vesicles, and multivesicular bodies (MVBs). AVi is preferred over AVi/d and AVd as the partner for fusion with endocytic structures ( Liou et al., 1997 ). Thus, fusion of autophagosomes with endosomes and lysosomes does not occur in a random order or in a strict sequential order, but in a multistage fashion ( Fig. 1 ). The composition and spatiotemporal distribution of endolysosomal compartments, which vary in different cell types and even under different growth conditions, may result in different preferential fusion combinations.
Fusion of AVs with functional early endosomes and MVBs is required for autophagy. Impairment of early endosome function by depleting COPI subunits (β′, β, or α) inhibits autophagosome maturation, resulting in accumulation of AVis and AVi/ds ( Razi et al., 2009 ). The accumulated AVs may have fused with very early endocytic compartments (before the appearance of the early endosomal protein EEA1), but are not acidic and lack lysosomal proteases ( Razi et al., 2009 ). Autophagosome maturation is also impaired when the formation of functional MVBs is disrupted by impairing the activities of ESCRT, which is required for the invagination of the endosomal membrane and the formation of intraluminal vesicles ( Filimonenko et al., 2007 ; Lee et al., 2007 ). Both autophagosomes and amphisomes accumulate in ESCRT-depleted cells, depending on the cell type and cell context ( Filimonenko et al., 2007 ; Lee et al., 2007 ; Rusten et al., 2007 ). ESCRT proteins may be involved in the recruitment of factors essential for fusion, such as Rab7, to modulate autophagosome maturation ( Urwin et al., 2010 ).
Live-cell imaging revealed that in addition to complete fusion, LC3-labeled autophagosomes/amphisomes can undergo kiss-and-run fusion with late endosomes (LEs)/lysosomes. In this process, a portion of the vesicle contents is delivered, but the two vesicles ultimately stay separate ( Jahreiss et al., 2008 ). The mechanisms underlying this multievent delivery remain unknown.
SNARE complexes mediate autophagosome–LE/lysosome fusion
Membrane fusion is driven by the formation of four-helix bundles from one of each of the Qa, Qb, Qc, and R SNARE proteins that are localized in opposing membranes ( Jahn and Scheller, 2006 ). Assembly of the trans-SNAREs is spatiotemporally controlled by the actions of Rab GTPase and tethering factors ( Fig. 2 A ).

SNAREs, tethers, and Rab proteins act in concert to mediate autophagosome–lysosome fusion. (A) Fusion of autophagosomes with LEs/lysosomes requires the concerted actions of SNARE proteins, Rab GTPases, and tethering factors. Amphisomes, which are single and membrane bound, also undergo fusion with LEs/lysosomes. (B) Two sets of cognate SNARE complexes (the autophagosomal STX17 [Qa]-SNAP29[Qbc]-endolysosomal VAMP7/8 [R] complex and the lysosomal STX7 [Qa]-SNAP29-endolysosomal YKT6 [R] complex) function in parallel with each other to mediate autophagosome–LE/lysosome fusion. ATG14 interacts with STX17 and promotes and stabilizes the assembly of the STX17 and SNAP29 complex. (C) Tethering proteins, including EPG5, HOPS, and PLEKHM1, simultaneously bind to ATG8s on autophagosomes and Rab7 on LEs/lysosomes to tether the autophagosomes/amphisomes with LEs/lysosomes for fusion. GRASP55 binds to Atg8s and LAMP2 on lysosomes. Lysosomal-localized BRUCE tethers autophagosomes through interaction with both ATG8s and STX17. HOPS has also been shown to target to autophagosomes via the Mon1-Ccz1-Rab7 module to promote autophagosome maturation. Rab7 is cycled between inactive GDP forms and active GTP forms by the Mon1-Ccz1 complex and the GAP protein Armus, respectively, during autophagosome maturation.
The STX17-SNAP29-VAMP8 complex and the YKT6-SNAP29-STX7 complex
Two cognate SNARE complexes act additively to mediate fusion of autophagosomes with LEs/lysosomes ( Fig. 2 B ). One complex consists of the autophagosomal-localized STX17 (Qa), SNAP29 (Qbc), and endolysosomal-localized VAMP8 (mammalian cells)/VAMP7 ( Drosophila melanogaster and Caenorhabditis elegans ; Itakura et al., 2012 ). Another complex is composed of the autophagosomal R-SNARE YKT6, SNAP29, and lysosomal-localized Qa SNARE STX7 ( Matsui et al., 2018 ). Depletion of these SNARE proteins causes accumulation of autophagosomes in different systems ( Sato et al., 2011 ; Itakura et al., 2012 ; Takáts et al., 2013 ; Matsui et al., 2018 ). YKT6 also forms a complex with STX17 and SNAP29 ( Matsui et al., 2018 ), which could be involved in fusion of autophagosomes with amphisomes/autolysosomes or homotypic fusion of autophagosomes and/or autolysosomes, depending on their respective localization. Multiple sets of SNARE complexes may promote the fusion efficiency or mediate fusion of autophagosomes with different populations of LEs/lysosomes containing differential levels of VAMP7/8 and STX7. Disassembly of the cis-SNARE complex on postfusion membranes, a process catalyzed by NSF and αSNAP, is essential for completion of the autophagosome–LE/lysosome fusion process ( Ishihara et al., 2001 ; Abada et al., 2017 ).
When and how are the STX17 and YKT6 proteins translocated to autophagic structures? STX17 and YKT6 are absent in IMs and are independently targeted to autophagosomes ( Matsui et al., 2018 ). The recruitment of STX17 is accompanied by autophagosome closure. STX17 also labels elliptical autophagosome-like structures in cells deficient in ESCRT-III components and the ATG8 conjugation system, suggesting that STX17 can be recruited before the completion of autophagosome closure ( Tsuboyama et al., 2016 ; Takahashi et al., 2018 ). The mechanisms mediating the targeting of STX17 and YKT6 to autophagosomes are distinct from their targeting to other membrane compartments. STX17 is a hairpin-tailed membrane-localized protein ( Itakura et al., 2012 ). The charged residues at the C-terminal flanking region are important for targeting of STX17 to the ER and mitochondria, but are dispensable for its localization to the autophagosome, while the glycine zipper-like motif in the trans-membrane domains is essential for integration of STX17 into the autophagosomal membrane but not for its targeting to the ER and mitochondria ( Itakura et al., 2012 ). In YKT6, the C-terminal cysteine residues for palmitoylation and farnesylation are essential for its Golgi localization but dispensable for its targeting to autophagosomes ( Matsui et al., 2018 ). The N-terminal longin domain is required and sufficient for the autophagosomal localization of YKT6 ( Matsui et al., 2018 ). Efficient translocation of STX17 to autophagosomes is facilitated by the small guanosine triphosphatase IRGM in human cells ( Kumar et al., 2018 ). IRGM interacts directly with STX17 and also with ATG8s via a noncanonical LIR region ( Kumar et al., 2018 ). The motifs involved in targeting STX17 and YKT6 to autophagosomes need to be released for subsequent assembly of trans-SNARE complexes. The LIR motif within the SNARE domain of STX17 binds to ATG8s ( Kumar et al., 2018 ). The longin domain in YKT6 binds to the intramolecular SNARE domain to inhibit SNARE complex assembly. However, ATG8s are not essential for STX17 and YKT6 recruitment. In cells depleted of all six ATG8s, or lacking the Atg8 conjugation system, STX17 and YKT6 still target to autophagosomes ( Tsuboyama et al., 2016 ). SNAP29 contains neither a trans-membrane domain nor a membrane-anchoring lipidation site, and thus it is recruited by interacting with STX17 or STX7.
VAMP7/8 are targeted to lysosomes via endocytic internalization and trafficking. The endolysosomal trafficking of VAMP7/8 is regulated by Rab21 and its guanine nucleotide exchange factor (GEF) protein MTMR13 ( Jean et al., 2015 ). Rab21 interacts with VAMP7/8, and the interaction is enhanced in response to starvation. In Rab21- or MTMR13-depleted cells, VAMP8 accumulates at early endosomes and leads to an increased number of autophagosomes ( Jean et al., 2015 ).
To maintain membrane identity and also to drive new rounds of fusion events, post-fusion SNAREs traffic back to their steady-state locations. Dissociation of STX17 from autolysosomes coincides with the collapse of the autophagosomal inner membrane and is independent of luminal acidification ( Tsuboyama et al., 2016 ). The molecular mechanisms sensing the integrity of the inner membrane and retrieval of SNAREs from autolysosomes have yet to be determined.
Tether proteins determine the fusion specificity of autophagosomes with LEs/lysosomes
Tethers enhance vesicle fusion specificity and efficiency. Tethering factors capture intracellular trafficking vesicles and bring them closer with targeting membranes, promoting and/or stabilizing the assembly of SNARE complexes ( Cai et al., 2007 ; Yu and Hughson, 2010 ). Tethers are recruited to specific membranes via their coordinated binding to Rab proteins, phospholipids, and SNAREs ( Cai et al., 2007 ; Yu and Hughson, 2010 ). Multiple tethering factors have been identified that localize on autophagosomes and/or LEs/lysosomes to promote autophagosome maturation ( Fig. 2 C ).
The essential autophagy gene EPG5 was identified from genetic screens in C. elegans ( Tian et al., 2010 ). EPG5 is localized on LEs/lysosomes under normal growth conditions and targets amphisomes/autolysosomes upon autophagy induction ( Wang et al., 2016 ). EGP5 is a Rab7 effector, and Rab7 mediates its membrane targeting. EPG5 recognizes autophagosomes by interacting with LC3 and also with assembled STX17-SNAP29. EPG5 stabilizes and facilitates the assembly of trans-SNARE proteins to facilitate fusion between autophagosomes and LEs/lysosomes ( Wang et al., 2016 ). EPG5 depletion causes formation of nondegradative autolysosomes ( Zhao et al., 2013a ; Wang et al., 2016 ). The membrane tethering and fusion-promoting activity of EPG5 has also been demonstrated in STX17-SNAP29-VAMP7–mediated fusion of reconstituted proteoliposomes ( Wang et al., 2016 ).
ATG14, a component of the VPS34 PI(3)P kinase complex, is also localized on sealed autophagosomes. ATG14 binds to the SNARE core domain of STX17 and stabilizes the STX17-SNAP29 complex to promote fusion of autophagosomes to endolysosomes ( Diao et al., 2015 ). In reconstituted proteoliposome systems, ATG14 contains tethering activity and also promotes membrane fusion mediated by the STX17-SNAP29-VAMP8 complex ( Diao et al., 2015 ).
Homotypic fusion and protein sorting (HOPS) complex
The multisubunit HOPS complex is involved in multiple fusion processes with LEs/lysosomes ( Yu and Hughson, 2010 ). HOPS is recruited to the membrane by binding to Rab7 and phospholipids such as PI(3)P ( Stroupe et al., 2006 ). HOPS facilitates membrane fusion by promoting the assembly of the trans-SNARE complex, and also by functioning as an SM protein to promote SNARE-mediated fusion ( Cai et al., 2007 ; Yu and Hughson, 2010 ). The HOPS complex is recruited to autophagosomes via its interaction with STX17 ( Jiang et al., 2014 ; Takáts et al., 2014 ) or by the Mon1-Ccz1-Rab7 module ( Hegedűs et al., 2016 ; Gao et al., 2018b ) to promote autophagosome maturation.
PLEKHM1 acts as a Rab7 effector and is localized on LEs/lysosomes ( Tabata et al., 2010 ). PLEKHM1 binds to ATG8 family proteins (see below), preferentially to GABARAPs, for autophagosome capture ( McEwan et al., 2015 ; Nguyen et al., 2016 ). PLEKHM1 recruits the HOPS complex to LEs/lysosomes and hence promotes the assembly of the SNARE complex ( McEwan et al., 2015 ). The function of PLEKHM1 in autophagosome maturation appears to be cell type specific. Depletion of PLEKHM1 causes no autophagy defect in A549 lung adenocarcinoma cells ( Tabata et al., 2010 ) or in C. elegans ( Wang et al., 2016 ).
Baculovirus IAP repeat-containing ubiquitin-conjugating enzyme (BRUCE)
BRUCE is localized on LEs/lysosomes and promotes autolysosome formation independent of its ubiquitin-conjugating activity ( Ebner et al., 2018 ). BRUCE interacts with ATG8s, preferentially with GABARAP and GABARAPL1, via noncanonical LIR-containing regions, and also interacts with STX17 and SNAP29 ( Ebner et al., 2018 ). Depletion of BRUCE leads to a defect in autophagosome–lysosome fusion ( Ebner et al., 2018 ).
The Golgi stacking protein GRASP55 is O -GlcNAc modified and locates in medial and trans-Golgi cisternae. Upon de- O -GlcNAcylation under glucose starvation, GRASP55 is targeted to autophagosomes and LEs/lysosomes ( Zhang et al., 2018 ). GRASP55 interacts via a LIR motif with Atg8 members, preferentially with LC3B, and simultaneously binds to LAMP2 on LEs/lysosomes to promote fusion ( Zhang et al., 2018 ). Depletion of GRASP55 inhibits autophagosome maturation, while expression of an O -GlcNAcylation–defective GRASP55 mutant accelerates autophagic flux ( Zhang et al., 2018 ).
Multiple tethering factors may act coordinately to enhance the efficiency and specificity of fusion, and mediate the fusion of autophagosomes with different populations of LEs/lysosomes. Different tethering factors may be differentially preferred by distinct SNARE complexes. Furthermore, different tethers may have distinct functions in mediating autophagosome–lysosome and amphisome–lysosome fusion in different cell types, tissues, and stress conditions, resulting in accumulation of AVs at different stages in a cell context–dependent manner. Tethers and SNARE proteins governing the fusion of autophagosomes with early endocytic vesicles remain to be determined.
ATG8 family members promote autophagosome maturation
ATG8 family members play multiple roles in autophagy. The single ATG8 in yeast is essential for IM expansion ( Nakatogawa et al., 2009 ; Feng et al., 2014 ). LGG-1 and LGG-2 in C. elegans , which belong to the GABARAP and LC3 families, respectively, act differentially in autophagy. LGG-1 depletion blocks autophagosome formation, while loss of LGG-2 causes the formation of smaller autophagosomes ( Wu et al., 2015 ). Mammalian cells contain six ATG8 homologues, in the LC3 subfamily (LC3A, LC3B, and LC3C) and the GABARAP subfamily (GABARAP, GABARAPL1, and GABARAPL2). The LC3 subfamily promotes elongation of IMs, while the GABARAP subfamily acts at the step of autophagosome closure ( Weidberg et al., 2010 ). In cells depleted of all six ATG8s, sealed autophagosomes can be formed, but the process is severely delayed and the autophagosomes are smaller ( Nguyen et al., 2016 ).
ATG8s, predominantly the GABARAP subfamily, play important roles in autophagosome maturation ( Nguyen et al., 2016 ; Pontano Vaites et al., 2017 ). Autophagosome–lysosome fusion is severely impaired by depletion of the three GABARAP members, and is almost completely blocked by depletion of all six ATG8s ( Nguyen et al., 2016 ; Pontano Vaites et al., 2017 ). Tethering factors, including EPG5, PLEKHM1, BRUCE, and GRASP55, interact with ATG8s for initial capture of autophagosomes. ATG8s also target HOPS to the autophagosome via direct interaction or indirectly by recruiting the Mon1-Ccz1-Rab7 module ( Manil-Ségalen et al., 2014 ; Gao et al., 2018b ). GABARAPs also target palmitoylated PI4KIIα to autophagosomes for generation of PI(4)P, which is critical for autophagosome–lysosome fusion ( Wang et al., 2015 ). LC3 and GABARAP possess membrane tethering and homotypic fusion activity ( Nakatogawa et al., 2007 ; Weidberg et al., 2011 ; Wu et al., 2015 ), which may directly contribute to fusion of autophagic structures. In contrast to cells depleted of all ATG8s, cells deficient in the ATG8 conjugation systems (e.g., ATG3, ATG5, and ATG7 KO cells) can still support the fusion of STX17-positive autophagosome-like structures with lysosomes ( Tsuboyama et al., 2016 ). Thus, lipidation of ATG8s may not be absolutely required for their role in autophagosome maturation. The ATG8 conjugation system is also important for efficient degradation of the inner autophagosomal membrane ( Tsuboyama et al., 2016 ).
Rabs, GEFs, and GTPase-activating proteins (GAPs) regulate autophagosome maturation
Rab GTPases specify membrane identity and regulate various processes of intracellular vesicle trafficking, including budding, uncoating, transport, and fusion, by recruiting effector proteins ( Stenmark, 2009 ; Mizuno-Yamasaki et al., 2012 ). Rab proteins cycle between GTP-bound active forms and GDP-bound inactive forms. The recruitment, activation, and function of Rab proteins at a specific membrane compartment is controlled by the concerted actions of GEFs and GAPs ( Mizuno-Yamasaki et al., 2012 ). GEFs target Rabs to a specific membrane and also catalyze exchange of GDP to GTP, which triggers recruitment of downstream effectors by Rabs ( Stenmark, 2009 ; Mizuno-Yamasaki et al., 2012 ). GAPs terminate Rab function in trafficking by accelerating conversion from the GTP- to the GDP-bound form ( Stenmark, 2009 ).
Rab7 is located on LEs/lysosomes ( Gutierrez et al., 2004 ; Jäger et al., 2004 ; Szatmári and Sass, 2014 ). In Rab7-knockdown cells, fusion of autophagosomes with early endosomes and MVBs still occurs, but fusion with lysosomes is blocked ( Gutierrez et al., 2004 ; Jäger et al., 2004 ; Eskelinen, 2005 ). Rab7 on LEs/lysosomes recruits tethering factors, including EPG5, PLEKHM1, and HOPS, to promote the assembly of trans-SNARE complexes for fusion ( Fig. 2 C ). Rab7 also functions on autophagosomes to promote maturation ( Hegedűs et al., 2016 ; Gao et al., 2018b ). The Mon1-Ccz1 complex, which acts as the Rab7 GEF and stabilizes its localization ( Nordmann et al., 2010 ), is targeted to autophagosomes via direct interaction with ATG8, which in turn recruits Rab7 and HOPS to promote autophagosome fusion with LEs/lysosomes ( Hegedűs et al., 2016 ; Pontano Vaites et al., 2017 ; Gao et al., 2018b ).
The membrane trafficking function of Rab7 is under tight spatiotemporal control. Rab7 inactivation triggers its release and facilitates completion of fusion. TBC/Rab GAP Armus (TBC1D2A), which is recruited to autophagosomes via interaction with LC3, promotes Rab7 cycling and thus facilitates autophagosome maturation ( Carroll et al., 2013 ). Armus depletion impairs Rab7 inactivation and delays autophagic flux ( Carroll et al., 2013 ).
Rab2, previously known to regulate vesicle trafficking at the ER and Golgi, is also involved in autophagosome maturation. Rab2 localizes to autophagosomes and recruits the HOPS complex to promote autophagosome maturation ( Fujita et al., 2017 ; Lőrincz et al., 2017 ). Rab2 depletion causes accumulation of autophagosomes in fly cells and amphisomes or autolysosomes in MEF cells ( Fujita et al., 2017 ; Lőrincz et al., 2017 ).
Vps34 PI(3)P kinase complexes modulate autophagosome maturation
Two Vps34 PI(3)P kinase complexes have been identified that act at different steps of the autophagy pathway. Both of them contain Beclin 1, hVps34, and hVps15, while UVRAG and Atg14L bind to Beclin 1 in a mutually exclusive manner ( Matsunaga et al., 2009 ). The Atg14L-containing Vps34 complex generates PI(3)P, which is essential for autophagosome formation ( Feng et al., 2014 ). Temporal turnover of PI(3)P from end-stage IMs and/or nascent autophagosomes triggers disassociation of PI(3)P-binding proteins and other Atg proteins and is a prerequisite for fusion of autophagosomes with endolysosomal compartments. Loss of function of the myotubularin PI(3)P phosphatase Ymr1 results in persistent association of Atg proteins with autophagosomes that fail to fuse with the vacuole in yeast ( Cebollero et al., 2012 ). Loss of function of the C. elegans myotubularin family PI(3)P phosphatase MTM-3 causes persistent association of ATG-18 and impairs autophagosome maturation ( Wu et al., 2014 ). PI(3)P, however, is also required for autophagosome maturation. PI(3)P on autophagosomes facilitates the recruitment of Mon1/Ccz1 or directly stabilizes Rab7 ( Hegedűs et al., 2016 ; Bas et al., 2018 ; Gao et al., 2018b ). Therefore, PI(3)P levels on autophagic structures must be deliberately controlled to ensure autophagosome maturation.
The UVRAG-containing PI(3)P kinase complex promotes autophagosome maturation ( Matsunaga et al., 2009 ). UVRAG is localized on early endosomal compartments under normal growth conditions ( Liang et al., 2008 ). Upon autophagy induction, UVRAG efficiently recruits the HOPS complex to AVs, thus stimulating Rab7 activity and autophagosome maturation ( Liang et al., 2008 ). UVRAG also activates the kinase function of the hVps34 complex ( Sun et al., 2010 ). Targeting of the UVRAG-Beclin1-Vps34 complex to autophagosomes is mediated by protein associated with UVRAG as autophagy enhancer (Pacer), which is recruited to autophagosomes by its interaction with STX17 and phosphoinositides ( Cheng et al., 2017 ). The role of the UVRAG-containing hVps34 complex in autophagosome maturation is negatively regulated by Rubicon ( Matsunaga et al., 2009 ; Zhong et al., 2009 ). Rubicon is highly enriched on early endosomes and directly interacts with Rab7, preferentially with GTP-bound Rab7 ( Sun et al., 2010 ; Tabata et al., 2010 ). Rubicon interacts with Rab7 and UVRAG in a mutually exclusive manner ( Sun et al., 2010 ). Rubicon may regulate autophagosome maturation by sequestration of UVRAG, thus preventing it from stimulating Vps34 kinase activity and binding HOPS ( Liang et al., 2010 ; Sun et al., 2010 ). The role of UVRAG in autophagosome maturation has been challenged by several recent studies. UVRAG is not present in the HOPS complex that mediates autophagosome maturation ( Jiang et al., 2014 ). The fusion of autophagosomes with lysosomes is largely unaffected by depletion of UVRAG in Drosophila and also in mammalian cells ( Itakura et al., 2008 ; Jiang et al., 2014 ; Takáts et al., 2014 ). The role of UVRAG in autophagosome maturation may depend on specific cell contexts, which differ in endolysosomal compartments, and also on experimental conditions, such as UVRAG overexpression.
The location of PI(3)P, Rabs, and tethering factors for autophagosome maturation
PI(3)P, Rabs, and tethering factors act coordinately to promote the assembly of the trans-SNARE complex. The sites where these different components function during autophagosome maturation have not been fully elucidated. Some components act at multiple steps of the autophagy pathway, and their late function during autophagosome maturation may be masked by their role in earlier steps of autophagosome formation. For example, Atg14 and PI(3)P act at both autophagosome formation and maturation ( Feng et al., 2014 ; Diao et al., 2015 ; Bas et al., 2018 ). The location at which these factors act and the stage of the AVs that accumulate after their depletion are mainly determined by their colocalization with LC3, STX17, and markers for LEs/lysosomes. LC3 and STX17 label nascent autophagosomes, amphisomes, and also autolysosomes (early-stage autolysosomes for STX17; Tsuboyama et al., 2016 ). More comprehensive analysis using a combination of assays, such as immuno-EM analysis, reporters for different vesicles, and tracers that are delivered to different stages of endocytic vesicles, is lacking for most of the factors involved in autophagosome maturation.
In vitro reconstitution of the fusion of autophagosomes with endosomes/lysosomes is useful to identify the essential factors and also to define the location of these factors during autophagosome maturation. Using isolated yeast vacuoles, autophagosomes, and cytosolic material derived from wild-type and mutants depleted of a specific autophagy gene, it was determined that Atg14 and Vps34 must be present in the cytosolic fraction for fusion to occur ( Bas et al., 2018 ). Ypt7 (yeast Rab7 homologue) acts on both autophagosomes and vacuoles to enhance fusion efficiency ( Bas et al., 2018 ). Consistent with this, purified autophagosomes carry Ypt7 and Mon1-Ccz1 on their surface ( Gao et al., 2018a ). Autophagosome-localized PI(3)P, generated by cytosolic Atg14/Vps34, may help to recruit and stabilize the Mon1-CCz1-Ypt7 complex on autophagosomes ( Bas et al., 2018 ; Gao et al., 2018a ). HOPS function is required on vacuoles but not on autophagosomes in the in vitro reconstitution assay ( Bas et al., 2018 ), although previous studies showed that HOPS also acts on autophagosomes to promote fusion ( Bas et al., 2018 ; Gao et al., 2018a ). The in vitro reconstitution assay also revealed that autophagosome–vacuole fusion requires the R-SNARE Ykt6 on the autophagosome and Vam3 (Qa), Vti1 (Qb), and Vam7 (Qc) on the vacuole ( Bas et al., 2018 ; Gao et al., 2018a ).
Lysosomal properties modulate fusion with autophagosomes and amphisomes
Lysosomes are the final destination for degradation of autophagosome-enclosed materials and also serve as a platform for mTORC1 activation to regulate autophagy induction. The acidic environment of lysosomes is not essential for autophagosome–lysosome fusion ( Mauvezin et al., 2015 ; Tsuboyama et al., 2016 ). However, the lysosomal membrane protein LAMP2 promotes fusion of lysosomes with autophagosomes/amphisomes ( Tanaka et al., 2000 ; Eskelinen, 2005 ). LAMP2 has been shown to interact with GRASP55 to facilitate the fusion process ( Zhang et al., 2018 ).
The lipid composition of the lysosomal membrane also regulates the fusion capability of lysosomes. Reduction or elevation of the lysosomal PI(3,5)P 2 level impairs autophagosome/amphisome–lysosome fusion ( de Lartigue et al., 2009 ; Ferguson et al., 2009 ). PI(3,5)P 2 is synthesized by the PI(3)P 5-kinase Fab1 (PIKfyve) and degraded by FIG4 (the PI(3,5)P 2 5-phosphatase)/VAC14 (the scaffold protein; Gary et al., 1998 , 2002 ). Fab1 is required for the maturation of amphisomes to autolysosomes ( Rusten et al., 2007 ). Mice deficient in Fig4 and Vac14 have a severely reduced cellular concentration of PI(3,5)Ps and also exhibit a defect in formation and recycling of autolysosomes ( Ferguson et al., 2009 ). The lysosomal-localized inositol polyphosphate-5-phosphatase E (INPP5E) down-regulates lysosomal PI(3,5)P 2 , which stabilizes actin filaments on the lysosome surface that are essential for lysosome-autophagosome fusion ( Hasegawa et al., 2016 ). Autophagosomes accumulate in INPP5E -depleted cells ( Hasegawa et al., 2016 ). Levels of lysosomal cholesterol also modulate autophagic flux. The myosin MYO1C facilitates recycling of cholesterol-enriched lipid rafts from intracellular compartments to the cell surface. Aberrant accumulation and distribution of cholesterol in the endolysosomal membrane in MYO1C -depleted cells or in lysosomal storage disorder cells are linked to reduced fusion of lysosomes with AVs ( Fraldi et al., 2010 ; Brandstaetter et al., 2014 ). Cholesterol-enriched endolysosomal membranes sequestrate assembled SNARE complexes, including Syntaxin 7 and VAMP7 and thus impair their sorting and recycling ( Fraldi et al., 2010 ). Other aspects of LEs/lysosomes influenced by a change in cholesterol levels, such as membrane rigidity and fluidity, may also impact autophagosome–lysosome fusion.
Lysosome positioning regulates fusion with autophagosomes and amphisomes
Lysosomes are most concentrated in the perinuclear region in nonpolarized cells and their position influences their function (reviewed in Pu et al., 2016 ). Autophagosomes are formed throughout the cytoplasm ( Jahreiss et al., 2008 ). Fusion is modulated by bidirectional movement of autophagosomes and lysosomes. Autophagosomes in the cell periphery move centripetally to fuse with juxtanuclear lysosomes or with lysosomes that undergo centrifugal transport. The centripetal movement (also known as minus end transport) of autophagosomes and lysosomes is driven by the motor protein dynein, while their centrifugal movement (plus end transport) is mediated by kinesin motors ( Jahreiss et al., 2008 ; Pu et al., 2016 ). Lysosome distribution is responsive to nutrient availability and influences mTORC1 activity. Starvation induces perinuclear clustering of lysosomes and inhibits mTORC1, while relocalization of lysosomes to the periphery by overexpression of kinesin activates mTORC1 ( Korolchuk et al., 2011 ). Movement of lysosomes, autophagosomes, and amphisomes affects their fusion frequency and also influences recruitment of tethering factors. The Rab7 effector FYCO1, which binds to LC3 and PI(3)P, mediates plus end–directed AV transport ( Pankiv et al., 2010 ). Depletion of FYCO1 leads to the perinuclear accumulation of clustered AVs. Transport and positioning of lysosomes and AVs are also regulated by the cholesterol-sensing Rab7 effector ORP1L ( Rocha et al., 2009 ; Wijdeven et al., 2016 ). ORP1L is localized on LEs/lysosomes, amphisomes, and autolysosomes and adopts different conformational states specified by the cholesterol level on these vesicles ( Rocha et al., 2009 ; Wijdeven et al., 2016 ). Under low cholesterol conditions, ORP1L forms a complex with VAPA on the ER, which removes the p150 Glued subunits of the dynein-dynactin motor from the Rab7-RILP complex and subsequently suppresses the centripetal movement of these vesicles ( Rocha et al., 2009 ; Wijdeven et al., 2016 ). Formation of the ORPIL-VAPA ER complex also inhibits the Rab7-RILP–mediated recruitment of PLEKHM1 and the HOPS complex, which impairs autophagosome maturation ( Wijdeven et al., 2016 ; Fig. 3 ).

Lysosome positioning modulates autophagosome–lysosome fusion efficiency. Center: Amphisome- and lysosome-localized ORP1L senses cholesterol levels. Under adequate cholesterol conditions, ORP1L binds to cholesterol on the membrane through its ORD domain, which further binds to Rab7/RILP for subsequent recruitment of PLEKHM1 and HOPS to facilitate autophagosome–endolysosome fusion. ORP1L also promotes the centripetal movement of amphisomes/autolysosomes through RILP-mediated recruitment of the motor protein dynein. Top: Under low-cholesterol conditions, the interaction of ORP1L with the ER protein VAPA tethers AVs to the ER, which inhibits dynein-mediated centripetal transport and also impairs autophagosome–lysosome fusion by reducing the recruitment of tether proteins. Bottom: The BORC complex increases the ARL8-dependent interaction with kinesins, leading to the centrifugal movement of lysosomes to the cell periphery for efficient fusion with autophagosomes. BORC also facilitates the fusion process by recruiting HOPS and promoting assembly of the STX17-SNAP29-VAMP8 SNAREs. PM, plasma membrane.
Lysosome dispersal increases the fusion of lysosomes with peripheral autophagosomes, which is also required for autophagic flux ( Fig. 3 ). The multisubunit BORC complex interacts with ARL8 on lysosomes to promote ARL8-dependent association to kinesins, resulting in lysosome movement toward the cell periphery. Depletion of BORC causes the juxtanuclear accumulation of lysosomes without affecting mTORC1 activity and reduces the frequency of encounter of peripheral autophagosomes with lysosomes ( Jia et al., 2017 ). BORC also promotes the ARL8-mediated recruitment of the HOPS complex and subsequently facilitates the assembly of STX17-SNAP29-VAMP8 for autophagosome–lysosome fusion ( Jia et al., 2017 ). ARL8b also directly binds to PLEKHM1 ( Marwaha et al., 2017 ). Therefore, the movement of autophagosomes and lysosomes affects the frequency of encounter and also the recruitment of fusion factors.
Nutrient status integrates into the autophagosome maturation machinery
Nutrient status is sensed by distinct signals that ultimately impinge on the Atg1 complex and the Vps34 complex to regulate autophagy induction. Starvation also promotes autophagosome maturation by enhancing the fusion process and lysosome biogenesis ( Fig. 4 ). Levels of UDP-GlcNAc, the precursor for post-translational O -GlcNAcylation, are highly responsive to the availability of glucose, fatty acids, uridine, and glutamine ( Slawson et al., 2010 ). Some components of the autophagosome fusion machinery are O -GlcNAc modified. SNAP29 is O -GlcNAcylated at multiple sites (Ser2, Ser61, Thr130, and Ser153 in mammals and Ser70, Ser134, Thr143, and Ser249 in C. elegans ), and levels of O- GlcNAcylated SNAP29 are reduced by nutrient starvation ( Guo et al., 2014 ). O -GlcNAc modification attenuates the interaction of SNAP29 with STX17 and VAMP8/7. Loss of function of OGT-1 ( O -GlcNAc transferase) or mutation of the O -GlcNAc sites in SNAP29 promotes formation of the SNAP29-containing SNARE complex and enhances autophagic flux ( Guo et al., 2014 ). De- O -GlcNAcylation of GRASP55 elicits its translocation to autophagosomes and LEs/lysosomes to promote fusion ( Zhang et al., 2018 ). The interaction of UVRAG with Rubicon is enhanced by mTORC1-mediated phosphorylation of UVRAG at Ser498 ( Kim et al., 2015 ). Dephosphorylation of UVRAG triggers the release of Rubicon, which then positively regulates Vps34 kinase activity and also interacts with the HOPS complex ( Kim et al., 2015 ). Therefore, post-translational modifications such as O -GlcNAcylation and phosphorylation serve as a mechanism for integrating nutrient availability with autophagosome maturation.

Nutrient status regulates autophagosome maturation through multiple mechanisms. Starvation decreases O -GlcNAcylation of SNAP29 and promotes its interaction with STX17 and VAMP8 to form trans-SNARE complexes for autophagosome–LE/lysosome fusion. Starvation-induced de- O -GlcNAcylation of GRASP55 causes its translocation to autophagosomes. De- O -GlcNAcylated GRASP55 simultaneously binds to LC3 and LAMP2 to facilitate autophagosome maturation. Inhibition of mTORC1 activity by starvation leads to dephosphorylation of UVRAG, which releases Rubicon and subsequently recruits HOPS to promote autophagosome fusion. The transcription of a network of genes involved in autophagosome–lysosome fusion is activated by the transcription factor TFEB and inhibited by the transcriptional repressor ZKSCAN3. Starvation triggers dephosphorylation of TFEB, resulting in its nuclear translocation. The translocation of ZKSCAN3 out of the nucleus is also promoted by nutrient deficiency.
Starvation and stress also promote nuclear localization and activity of the bHLH-leucine zipper transcription factors TFEB and/or TFE3, which activate a network of genes involved in autophagosome and lysosome biogenesis and also autophagosome–lysosome fusion ( Raben and Puertollano, 2016 ). Phosphorylation controls the cytoplasmic-nuclear shuttling of TFEB/TFE3; the nonphosphorylated proteins translocate into the nucleus. Distinct nutrient stimuli are transduced via different signaling pathways to mediate phosphorylation of TFEB/TFE3. Phosphorylation of TFEB at Ser 142 via extracellular signal-regulated kinase 2 retains TFEB in the cytosol ( Settembre et al., 2011 ). mTORC1, which senses amino acid status and other stresses, phosphorylates TFEB at Ser211 and TFE3 at Ser321, creating binding sites for 14-3-3 proteins that mediate cytosolic retention ( Settembre et al., 2012 ; Raben and Puertollano, 2016 ). Under starvation conditions, TFEB/TFE3 are dephosphorylated by calcineurin, a phosphatase activated by starvation-triggered lysosomal calcium release ( Medina et al., 2015 ). PKC also controls nuclear translocation and activation of TFEB by regulating GSK3β activity ( Li et al., 2016 ). GSK3β phosphorylates TFEB at Ser 134 and Ser 138 ( Li et al., 2016 ).
The zinc-finger family DNA-binding protein ZKSCAN3 acts in opposition to TFEB/TFE3 to repress transcription of a set of genes involved in lysosome biogenesis/function and autophagosome–lysosome fusion ( Chauhan et al., 2013 ). Subcellular localization of ZKSCAN3 is modulated by nutrient availability and stress ( Chauhan et al., 2013 ). PKC activates JNK and p38 MAPK to phosphorylate ZKSCAN3 at Thr 153, which triggers ZKSCAN3 translocation out of the nucleus ( Li et al., 2016 ).
Autophagosome maturation and neurodegenerative diseases
In neurons, distinct from other cell types, autophagosome formation and maturation exhibit spatiotemporal features. Autophagosomes are preferentially generated at the distal tip of the axon, and then undergo retrograde trafficking through the axon to the soma, accompanied by stepwise maturation into autolysosomes ( Maday et al., 2012 ). Autophagosome formation in neurons requires the same set of autophagy proteins, although the membrane source for expansion of IMs may be distinct ( Maday and Holzbaur, 2014 ). The STX17-containing SNARE complex, Rab7, EPG5, and the HOPS complex are involved in autophagosome maturation ( Bains et al., 2011 ; Peng et al., 2012 ; Takáts et al., 2013 ; Zhao et al., 2013a ; Cheng et al., 2015 ; Zhen and Li, 2015 ). Neurodegenerative diseases, such as Huntington’s disease, Parkinson’s disease, and amyotrophic lateral sclerosis (ALS), show impaired formation and function of autolysosomes, resulting in gradual accumulation of AVs in affected neurons and eventual cell death ( Nixon et al., 2008 ). Mice with neural-specific depletion of genes essential for autophagosome formation, including Atg5 , Atg7 , Fip200, and Ei24 , exhibit massive neuronal death ( Hara et al., 2006 ; Komatsu et al., 2006 ; Liang et al., 2010 ; Zhao et al., 2012 ). However, these knockout mice fail to recapitulate the features of neurodegenerative diseases, including accumulation of AVs and damage of only a certain population of neurons ( Hardy and Gwinn-Hardy, 1998 ).
Loss of function of EPG5 causes accumulation of nondegradative AVs ( Zhao et al., 2013a ). Epg5 KO mice develop age-dependent selective neuronal damage and exhibit characteristics of ALS ( Zhao et al., 2013a ). The pyramidal neurons in the fifth layer of the cerebral cortices and motor neurons in the anterior horn of the spinal cord are gradually lost in Epg5 KO mice, resulting in muscle atrophy and muscle denervation ( Zhao et al., 2013a ). Epg5 KO mice also display features of retinitis pigmentosa ( Miao et al., 2016 ). Depletion of EPG5 impairs autophagy in various types of neurons; the mechanism underlying damage of only a specific population of neurons has yet to be determined ( Zhao et al., 2013a ). Recent human genetic studies revealed that recessive EPG5 mutations are causatively linked with the multisystem disorder Vici syndrome, characterized by neurodegenerative features as displayed in Epg5 KO mice, and also a defective immune response ( Cullup et al., 2013 ; Zhao et al., 2013b ). Mutations in the ESCRT-III complex subunit CHMP2B are associated with frontotemporal dementia and ALS ( Skibinski et al., 2005 ; Parkinson et al., 2006 ). Different factors may act differentially in autophagosome maturation in distinct types of neurons, so that their loss of function causes autophagy defects of variable severity. Accumulation of AVs may interfere with endocytic trafficking, thus contributing to selective damage of a certain population of neurons.
Numerous factors involved in fusion of autophagosomes with LEs/lysosomes have been identified, including Rabs, tethers, and SNARE proteins; however, many fundamental questions remain to be answered. What is the molecular machinery that mediates the fusion of the earliest endocytic vesicles with autophagosomes? How do different tethering factors act coordinately in autophagosome maturation? Do different tethering factors mediate fusion of autophagosomes with distinct populations of endocytic vesicles? Is the homotypic fusion of autophagosomes/amphisomes/autolysosomes mediated by the same set of SNARE proteins as heterotypic fusion? How is the fusion of autophagosomes with other vesicles, such as recycling endosomes and secretory vesicles, prevented? In EPG5 -deficient cells, autophagosomes undergo nonspecific fusion with other vesicles ( Zhao et al., 2013a ). How does the accumulation of AVs affect other endocytic trafficking and recycling processes? Understanding the mechanisms underlying autophagosome maturation will provide more insights into the pathogenesis of relevant human diseases as well as providing more therapeutic clues for treatment.
Acknowledgments
We are grateful to Dr. Isabel Hanson for editing the manuscript.
Work in the authors’ laboratory was supported by the National Natural Science Foundation of China (grants 31630048, 31421002, and 31561143001), the Strategic Priority Research Program of the Chinese Academy of Sciences (grant XDB19000000), the Key Research Program of Frontier Sciences, Chinese Academy of Sciences (grant QYZDY-SSW-SMC006), and the Orphan Disease Center’s Million Dollar Bike Ride pilot grant program (MDBR-18-104-BPAN).
The authors declare no competing financial interests.
Author contributions: Y.G. Zhao and H. Zhang wrote and edited the manuscript.
- Abada A., Levin-Zaidman S., Porat Z., Dadosh T., and Elazar Z.. 2017. SNARE priming is essential for maturation of autophagosomes but not for their formation . Proc. Natl. Acad. Sci. USA. 114 :12749–12754. 10.1073/pnas.1705572114 [ Europe PMC free article ] [ Abstract ] [ CrossRef ] [ Google Scholar ]
- Anding A.L., and Baehrecke E.H.. 2017. Cleaning House: Selective Autophagy of Organelles . Dev. Cell. 41 :10–22. 10.1016/j.devcel.2017.02.016 [ Europe PMC free article ] [ Abstract ] [ CrossRef ] [ Google Scholar ]
- Axe E.L., Walker S.A., Manifava M., Chandra P., Roderick H.L., Habermann A., Griffiths G., and Ktistakis N.T.. 2008. Autophagosome formation from membrane compartments enriched in phosphatidylinositol 3-phosphate and dynamically connected to the endoplasmic reticulum . J. Cell Biol. 182 :685–701. 10.1083/jcb.200803137 [ Europe PMC free article ] [ Abstract ] [ CrossRef ] [ Google Scholar ]
- Bains M., Zaegel V., Mize-Berge J., and Heidenreich K.A.. 2011. IGF-I stimulates Rab7-RILP interaction during neuronal autophagy . Neurosci. Lett. 488 :112–117. 10.1016/j.neulet.2010.09.018 [ Europe PMC free article ] [ Abstract ] [ CrossRef ] [ Google Scholar ]
- Bas L., Papinski D., Licheva M., Torggler R., Rohringer S., Schuschnig M., and Kraft C.. 2018. Reconstitution reveals Ykt6 as the autophagosomal SNARE in autophagosome-vacuole fusion . J. Cell Biol. 217 :3656–3669. 10.1083/jcb.201804028 [ Europe PMC free article ] [ Abstract ] [ CrossRef ] [ Google Scholar ]
- Brandstaetter H., Kishi-Itakura C., Tumbarello D.A., Manstein D.J., and Buss F.. 2014. Loss of functional MYO1C/myosin 1c, a motor protein involved in lipid raft trafficking, disrupts autophagosome-lysosome fusion . Autophagy. 10 :2310–2323. 10.4161/15548627.2014.984272 [ Europe PMC free article ] [ Abstract ] [ CrossRef ] [ Google Scholar ]
- Cai H., Reinisch K., and Ferro-Novick S.. 2007. Coats, tethers, Rabs, and SNAREs work together to mediate the intracellular destination of a transport vesicle . Dev. Cell. 12 :671–682. 10.1016/j.devcel.2007.04.005 [ Abstract ] [ CrossRef ] [ Google Scholar ]
- Carroll B., Mohd-Naim N., Maximiano F., Frasa M.A., McCormack J., Finelli M., Thoresen S.B., Perdios L., Daigaku R., Francis R.E., et al. . 2013. The TBC/RabGAP Armus coordinates Rac1 and Rab7 functions during autophagy . Dev. Cell. 25 :15–28. 10.1016/j.devcel.2013.03.005 [ Europe PMC free article ] [ Abstract ] [ CrossRef ] [ Google Scholar ]
- Cebollero E., van der Vaart A., Zhao M., Rieter E., Klionsky D.J., Helms J.B., and Reggiori F.. 2012. Phosphatidylinositol-3-phosphate clearance plays a key role in autophagosome completion . Curr. Biol. 22 :1545–1553. 10.1016/j.cub.2012.06.029 [ Europe PMC free article ] [ Abstract ] [ CrossRef ] [ Google Scholar ]
- Chauhan S., Goodwin J.G., Chauhan S., Manyam G., Wang J., Kamat A.M., and Boyd D.D.. 2013. ZKSCAN3 is a master transcriptional repressor of autophagy . Mol. Cell. 50 :16–28. 10.1016/j.molcel.2013.01.024 [ Europe PMC free article ] [ Abstract ] [ CrossRef ] [ Google Scholar ]
- Cheng X.T., Zhou B., Lin M.Y., Cai Q., and Sheng Z.H.. 2015. Axonal autophagosomes recruit dynein for retrograde transport through fusion with late endosomes . J. Cell Biol. 209 :377–386. 10.1083/jcb.201412046 [ Europe PMC free article ] [ Abstract ] [ CrossRef ] [ Google Scholar ]
- Cheng X., Ma X., Ding X., Li L., Jiang X., Shen Z., Chen S., Liu W., Gong W., and Sun Q.. 2017. Pacer Mediates the Function of Class III PI3K and HOPS Complexes in Autophagosome Maturation by Engaging Stx17 . Mol. Cell. 65 :1029–1043.e5. 10.1016/j.molcel.2017.02.010 [ Abstract ] [ CrossRef ] [ Google Scholar ]
- Cheng X.T., Xie Y.X., Zhou B., Huang N., Farfel-Becker T., and Sheng Z.H.. 2018. Characterization of LAMP1-labeled nondegradative lysosomal and endocytic compartments in neurons . J. Cell Biol. 217 :3127–3139. 10.1083/jcb.201711083 [ Europe PMC free article ] [ Abstract ] [ CrossRef ] [ Google Scholar ]
- Cullup T., Kho A.L., Dionisi-Vici C., Brandmeier B., Smith F., Urry Z., Simpson M.A., Yau S., Bertini E., McClelland V., et al. . 2013. Recessive mutations in EPG5 cause Vici syndrome, a multisystem disorder with defective autophagy . Nat. Genet. 45 :83–87. 10.1038/ng.2497 [ Europe PMC free article ] [ Abstract ] [ CrossRef ] [ Google Scholar ]
- de Lartigue J., Polson H., Feldman M., Shokat K., Tooze S.A., Urbé S., and Clague M.J.. 2009. PIKfyve regulation of endosome-linked pathways . Traffic. 10 :883–893. 10.1111/j.1600-0854.2009.00915.x [ Europe PMC free article ] [ Abstract ] [ CrossRef ] [ Google Scholar ]
- Diao J., Liu R., Rong Y., Zhao M., Zhang J., Lai Y., Zhou Q., Wilz L.M., Li J., Vivona S., et al. . 2015. ATG14 promotes membrane tethering and fusion of autophagosomes to endolysosomes . Nature. 520 :563–566. 10.1038/nature14147 [ Europe PMC free article ] [ Abstract ] [ CrossRef ] [ Google Scholar ]
- Dunn W.A., Jr 1990. Studies on the mechanisms of autophagy: maturation of the autophagic vacuole . J. Cell Biol. 110 :1935–1945. 10.1083/jcb.110.6.1935 [ Europe PMC free article ] [ Abstract ] [ CrossRef ] [ Google Scholar ]
- Ebner P., Poetsch I., Deszcz L., Hoffmann T., Zuber J., and Ikeda F.. 2018. The IAP family member BRUCE regulates autophagosome-lysosome fusion . Nat. Commun. 9 :599 10.1038/s41467-018-02823-x [ Europe PMC free article ] [ Abstract ] [ CrossRef ] [ Google Scholar ]
- Eskelinen E.L. 2005. Maturation of autophagic vacuoles in Mammalian cells . Autophagy. 1 :1–10. 10.4161/auto.1.1.1270 [ Abstract ] [ CrossRef ] [ Google Scholar ]
- Feng Y., He D., Yao Z., and Klionsky D.J.. 2014. The machinery of macroautophagy . Cell Res. 24 :24–41. 10.1038/cr.2013.168 [ Europe PMC free article ] [ Abstract ] [ CrossRef ] [ Google Scholar ]
- Ferguson C.J., Lenk G.M., and Meisler M.H.. 2009. Defective autophagy in neurons and astrocytes from mice deficient in PI(3,5)P2 . Hum. Mol. Genet. 18 :4868–4878. 10.1093/hmg/ddp460 [ Europe PMC free article ] [ Abstract ] [ CrossRef ] [ Google Scholar ]
- Filimonenko M., Stuffers S., Raiborg C., Yamamoto A., Malerød L., Fisher E.M.C., Isaacs A., Brech A., Stenmark H., and Simonsen A.. 2007. Functional multivesicular bodies are required for autophagic clearance of protein aggregates associated with neurodegenerative disease . J. Cell Biol. 179 :485–500. 10.1083/jcb.200702115 [ Europe PMC free article ] [ Abstract ] [ CrossRef ] [ Google Scholar ]
- Fraldi A., Annunziata F., Lombardi A., Kaiser H.J., Medina D.L., Spampanato C., Fedele A.O., Polishchuk R., Sorrentino N.C., Simons K., and Ballabio A.. 2010. Lysosomal fusion and SNARE function are impaired by cholesterol accumulation in lysosomal storage disorders . EMBO J. 29 :3607–3620. 10.1038/emboj.2010.237 [ Europe PMC free article ] [ Abstract ] [ CrossRef ] [ Google Scholar ] Retracted
- Fujita N., Huang W., Lin T.H., Groulx J.F., Jean S., Nguyen J., Kuchitsu Y., Koyama-Honda I., Mizushima N., Fukuda M., and Kiger A.A.. 2017. Genetic screen in Drosophila muscle identifies autophagy-mediated T-tubule remodeling and a Rab2 role in autophagy . eLife. 6 :e23367 10.7554/eLife.23367 [ Europe PMC free article ] [ Abstract ] [ CrossRef ] [ Google Scholar ]
- Gao J., Langemeyer L., Kümmel D., Reggiori F., and Ungermann C.. 2018b Molecular mechanism to target the endosomal Mon1-Ccz1 GEF complex to the pre-autophagosomal structure . eLife. 7 :e31145 10.7554/eLife.31145 [ Europe PMC free article ] [ Abstract ] [ CrossRef ] [ Google Scholar ]
- Gao J., Reggiori F., and Ungermann C.. 2018a A novel in vitro assay reveals SNARE topology and the role of Ykt6 in autophagosome fusion with vacuoles . J. Cell Biol. 217 :3670–3682. 10.1083/jcb.201804039 [ Europe PMC free article ] [ Abstract ] [ CrossRef ] [ Google Scholar ]
- Gary J.D., Wurmser A.E., Bonangelino C.J., Weisman L.S., and Emr S.D.. 1998. Fab1p is essential for PtdIns(3)P 5-kinase activity and the maintenance of vacuolar size and membrane homeostasis . J. Cell Biol. 143 :65–79. 10.1083/jcb.143.1.65 [ Europe PMC free article ] [ Abstract ] [ CrossRef ] [ Google Scholar ]
- Gary J.D., Sato T.K., Stefan C.J., Bonangelino C.J., Weisman L.S., and Emr S.D.. 2002. Regulation of Fab1 phosphatidylinositol 3-phosphate 5-kinase pathway by Vac7 protein and Fig4, a polyphosphoinositide phosphatase family member . Mol. Biol. Cell. 13 :1238–1251. 10.1091/mbc.01-10-0498 [ Europe PMC free article ] [ Abstract ] [ CrossRef ] [ Google Scholar ]
- Ge L., Melville D., Zhang M., and Schekman R.. 2013. The ER-Golgi intermediate compartment is a key membrane source for the LC3 lipidation step of autophagosome biogenesis . eLife. 2 :e00947 10.7554/eLife.00947 [ Europe PMC free article ] [ Abstract ] [ CrossRef ] [ Google Scholar ]
- Gómez-Sánchez R., Rose J., Guimarães R., Mari M., Papinski D., Rieter E., Geerts W.J., Hardenberg R., Kraft C., Ungermann C., and Reggiori F.. 2018. Atg9 establishes Atg2-dependent contact sites between the endoplasmic reticulum and phagophores . J. Cell Biol. 217 :2743–2763. 10.1083/jcb.201710116 [ Europe PMC free article ] [ Abstract ] [ CrossRef ] [ Google Scholar ]
- Graef M., Friedman J.R., Graham C., Babu M., and Nunnari J.. 2013. ER exit sites are physical and functional core autophagosome biogenesis components . Mol. Biol. Cell. 24 :2918–2931. 10.1091/mbc.e13-07-0381 [ Europe PMC free article ] [ Abstract ] [ CrossRef ] [ Google Scholar ]
- Guo B., Liang Q., Li L., Hu Z., Wu F., Zhang P., Ma Y., Zhao B., Kovács A.L., Zhang Z., et al. . 2014. O-GlcNAc-modification of SNAP-29 regulates autophagosome maturation . Nat. Cell Biol. 16 :1215–1226. 10.1038/ncb3066 [ Abstract ] [ CrossRef ] [ Google Scholar ]
- Gutierrez M.G., Munafó D.B., Berón W., and Colombo M.I.. 2004. Rab7 is required for the normal progression of the autophagic pathway in mammalian cells . J. Cell Sci. 117 :2687–2697. 10.1242/jcs.01114 [ Abstract ] [ CrossRef ] [ Google Scholar ]
- Hailey D.W., Rambold A.S., Satpute-Krishnan P., Mitra K., Sougrat R., Kim P.K., and Lippincott-Schwartz J.. 2010. Mitochondria supply membranes for autophagosome biogenesis during starvation . Cell. 141 :656–667. 10.1016/j.cell.2010.04.009 [ Europe PMC free article ] [ Abstract ] [ CrossRef ] [ Google Scholar ]
- Hara T., Nakamura K., Matsui M., Yamamoto A., Nakahara Y., Suzuki-Migishima R., Yokoyama M., Mishima K., Saito I., Okano H., and Mizushima N.. 2006. Suppression of basal autophagy in neural cells causes neurodegenerative disease in mice . Nature. 441 :885–889. 10.1038/nature04724 [ Abstract ] [ CrossRef ] [ Google Scholar ]
- Hardy J., and Gwinn-Hardy K.. 1998. Genetic classification of primary neurodegenerative disease . Science. 282 :1075–1079. 10.1126/science.282.5391.1075 [ Abstract ] [ CrossRef ] [ Google Scholar ]
- Hasegawa J., Iwamoto R., Otomo T., Nezu A., Hamasaki M., and Yoshimori T.. 2016. Autophagosome-lysosome fusion in neurons requires INPP5E, a protein associated with Joubert syndrome . EMBO J. 35 :1853–1867. 10.15252/embj.201593148 [ Europe PMC free article ] [ Abstract ] [ CrossRef ] [ Google Scholar ]
- Hayashi-Nishino M., Fujita N., Noda T., Yamaguchi A., Yoshimori T., and Yamamoto A.. 2009. A subdomain of the endoplasmic reticulum forms a cradle for autophagosome formation . Nat. Cell Biol. 11 :1433–1437. 10.1038/ncb1991 [ Abstract ] [ CrossRef ] [ Google Scholar ]
- Hegedűs K., Takáts S., Boda A., Jipa A., Nagy P., Varga K., Kovács A.L., and Juhász G.. 2016. The Ccz1-Mon1-Rab7 module and Rab5 control distinct steps of autophagy . Mol. Biol. Cell. 27 :3132–3142. 10.1091/mbc.e16-03-0205 [ Europe PMC free article ] [ Abstract ] [ CrossRef ] [ Google Scholar ]
- Ishihara N., Hamasaki M., Yokota S., Suzuki K., Kamada Y., Kihara A., Yoshimori T., Noda T., and Ohsumi Y.. 2001. Autophagosome requires specific early Sec proteins for its formation and NSF/SNARE for vacuolar fusion . Mol. Biol. Cell. 12 :3690–3702. 10.1091/mbc.12.11.3690 [ Europe PMC free article ] [ Abstract ] [ CrossRef ] [ Google Scholar ]
- Itakura E., and Mizushima N.. 2010. Characterization of autophagosome formation site by a hierarchical analysis of mammalian Atg proteins . Autophagy. 6 :764–776. 10.4161/auto.6.6.12709 [ Europe PMC free article ] [ Abstract ] [ CrossRef ] [ Google Scholar ]
- Itakura E., Kishi C., Inoue K., and Mizushima N.. 2008. Beclin 1 forms two distinct phosphatidylinositol 3-kinase complexes with mammalian Atg14 and UVRAG . Mol. Biol. Cell. 19 :5360–5372. 10.1091/mbc.e08-01-0080 [ Europe PMC free article ] [ Abstract ] [ CrossRef ] [ Google Scholar ]
- Itakura E., Kishi-Itakura C., and Mizushima N.. 2012. The hairpin-type tail-anchored SNARE syntaxin 17 targets to autophagosomes for fusion with endosomes/lysosomes . Cell. 151 :1256–1269. 10.1016/j.cell.2012.11.001 [ Abstract ] [ CrossRef ] [ Google Scholar ]
- Jäger S., Bucci C., Tanida I., Ueno T., Kominami E., Saftig P., and Eskelinen E.L.. 2004. Role for Rab7 in maturation of late autophagic vacuoles . J. Cell Sci. 117 :4837–4848. 10.1242/jcs.01370 [ Abstract ] [ CrossRef ] [ Google Scholar ]
- Jahn R., and Scheller R.H.. 2006. SNAREs--engines for membrane fusion . Nat. Rev. Mol. Cell Biol. 7 :631–643. 10.1038/nrm2002 [ Abstract ] [ CrossRef ] [ Google Scholar ]
- Jahreiss L., Menzies F.M., and Rubinsztein D.C.. 2008. The itinerary of autophagosomes: from peripheral formation to kiss-and-run fusion with lysosomes . Traffic. 9 :574–587. 10.1111/j.1600-0854.2008.00701.x [ Europe PMC free article ] [ Abstract ] [ CrossRef ] [ Google Scholar ]
- Jean S., Cox S., Nassari S., and Kiger A.A.. 2015. Starvation-induced MTMR13 and RAB21 activity regulates VAMP8 to promote autophagosome-lysosome fusion . EMBO Rep. 16 :297–311. 10.15252/embr.201439464 [ Europe PMC free article ] [ Abstract ] [ CrossRef ] [ Google Scholar ]
- Jia R., Guardia C.M., Pu J., Chen Y., and Bonifacino J.S.. 2017. BORC coordinates encounter and fusion of lysosomes with autophagosomes . Autophagy. 13 :1648–1663. 10.1080/15548627.2017.1343768 [ Europe PMC free article ] [ Abstract ] [ CrossRef ] [ Google Scholar ]
- Jiang P., Nishimura T., Sakamaki Y., Itakura E., Hatta T., Natsume T., and Mizushima N.. 2014. The HOPS complex mediates autophagosome-lysosome fusion through interaction with syntaxin 17 . Mol. Biol. Cell. 25 :1327–1337. 10.1091/mbc.e13-08-0447 [ Europe PMC free article ] [ Abstract ] [ CrossRef ] [ Google Scholar ]
- Karanasios E., Walker S.A., Okkenhaug H., Manifava M., Hummel E., Zimmermann H., Ahmed Q., Domart M.C., Collinson L., and Ktistakis N.T.. 2016. Autophagy initiation by ULK complex assembly on ER tubulovesicular regions marked by ATG9 vesicles . Nat. Commun. 7 :12420 10.1038/ncomms12420 [ Europe PMC free article ] [ Abstract ] [ CrossRef ] [ Google Scholar ]
- Kim Y.M., Jung C.H., Seo M., Kim E.K., Park J.M., Bae S.S., and Kim D.H.. 2015. mTORC1 phosphorylates UVRAG to negatively regulate autophagosome and endosome maturation . Mol. Cell. 57 :207–218. 10.1016/j.molcel.2014.11.013 [ Europe PMC free article ] [ Abstract ] [ CrossRef ] [ Google Scholar ]
- Komatsu M., Waguri S., Chiba T., Murata S., Iwata J., Tanida I., Ueno T., Koike M., Uchiyama Y., Kominami E., and Tanaka K.. 2006. Loss of autophagy in the central nervous system causes neurodegeneration in mice . Nature. 441 :880–884. 10.1038/nature04723 [ Abstract ] [ CrossRef ] [ Google Scholar ]
- Korolchuk V.I., Saiki S., Lichtenberg M., Siddiqi F.H., Roberts E.A., Imarisio S., Jahreiss L., Sarkar S., Futter M., Menzies F.M., et al. . 2011. Lysosomal positioning coordinates cellular nutrient responses . Nat. Cell Biol. 13 :453–460. 10.1038/ncb2204 [ Europe PMC free article ] [ Abstract ] [ CrossRef ] [ Google Scholar ]
- Kotani T., Kirisako H., Koizumi M., Ohsumi Y., and Nakatogawa H.. 2018. The Atg2-Atg18 complex tethers pre-autophagosomal membranes to the endoplasmic reticulum for autophagosome formation . Proc. Natl. Acad. Sci. USA. 115 :10363–10368. 10.1073/pnas.1806727115 [ Europe PMC free article ] [ Abstract ] [ CrossRef ] [ Google Scholar ]
- Kumar S., Jain A., Farzam F., Jia J., Gu Y., Choi S.W., Mudd M.H., Claude-Taupin A., Wester M.J., Lidke K.A., et al. . 2018. Mechanism of Stx17 recruitment to autophagosomes via IRGM and mammalian Atg8 proteins . J. Cell Biol. 217 :997–1013. 10.1083/jcb.201708039 [ Europe PMC free article ] [ Abstract ] [ CrossRef ] [ Google Scholar ]
- Lamb C.A., Yoshimori T., and Tooze S.A.. 2013. The autophagosome: origins unknown, biogenesis complex . Nat. Rev. Mol. Cell Biol. 14 :759–774. 10.1038/nrm3696 [ Abstract ] [ CrossRef ] [ Google Scholar ]
- Lee J.A., Beigneux A., Ahmad S.T., Young S.G., and Gao F.B.. 2007. ESCRT-III dysfunction causes autophagosome accumulation and neurodegeneration . Curr. Biol. 17 :1561–1567. 10.1016/j.cub.2007.07.029 [ Abstract ] [ CrossRef ] [ Google Scholar ]
- Li Y., Xu M., Ding X., Yan C., Song Z., Chen L., Huang X., Wang X., Jian Y., Tang G., et al. . 2016. Protein kinase C controls lysosome biogenesis independently of mTORC1 . Nat. Cell Biol. 18 :1065–1077. 10.1038/ncb3407 [ Abstract ] [ CrossRef ] [ Google Scholar ]
- Liang C., Lee J.S., Inn K.S., Gack M.U., Li Q., Roberts E.A., Vergne I., Deretic V., Feng P., Akazawa C., and Jung J.U.. 2008. Beclin1-binding UVRAG targets the class C Vps complex to coordinate autophagosome maturation and endocytic trafficking . Nat. Cell Biol. 10 :776–787. 10.1038/ncb1740 [ Europe PMC free article ] [ Abstract ] [ CrossRef ] [ Google Scholar ]
- Liang C.C., Wang C., Peng X., Gan B., and Guan J.L.. 2010. Neural-specific deletion of FIP200 leads to cerebellar degeneration caused by increased neuronal death and axon degeneration . J. Biol. Chem. 285 :3499–3509. 10.1074/jbc.M109.072389 [ Europe PMC free article ] [ Abstract ] [ CrossRef ] [ Google Scholar ]
- Liou W., Geuze H.J., Geelen M.J., and Slot J.W.. 1997. The autophagic and endocytic pathways converge at the nascent autophagic vacuoles . J. Cell Biol. 136 :61–70. 10.1083/jcb.136.1.61 [ Europe PMC free article ] [ Abstract ] [ CrossRef ] [ Google Scholar ]
- Lőrincz P., Tóth S., Benkő P., Lakatos Z., Boda A., Glatz G., Zobel M., Bisi S., Hegedűs K., Takáts S., et al. . 2017. Rab2 promotes autophagic and endocytic lysosomal degradation . J. Cell Biol. 216 :1937–1947. 10.1083/jcb.201611027 [ Europe PMC free article ] [ Abstract ] [ CrossRef ] [ Google Scholar ]
- Maday S., and Holzbaur E.L.. 2014. Autophagosome biogenesis in primary neurons follows an ordered and spatially regulated pathway . Dev. Cell. 30 :71–85. 10.1016/j.devcel.2014.06.001 [ Europe PMC free article ] [ Abstract ] [ CrossRef ] [ Google Scholar ]
- Maday S., Wallace K.E., and Holzbaur E.L.. 2012. Autophagosomes initiate distally and mature during transport toward the cell soma in primary neurons . J. Cell Biol. 196 :407–417. 10.1083/jcb.201106120 [ Europe PMC free article ] [ Abstract ] [ CrossRef ] [ Google Scholar ]
- Manil-Ségalen M., Lefebvre C., Jenzer C., Trichet M., Boulogne C., Satiat-Jeunemaitre B., and Legouis R.. 2014. The C. elegans LC3 acts downstream of GABARAP to degrade autophagosomes by interacting with the HOPS subunit VPS39 . Dev. Cell. 28 :43–55. 10.1016/j.devcel.2013.11.022 [ Abstract ] [ CrossRef ] [ Google Scholar ]
- Marwaha R., Arya S.B., Jagga D., Kaur H., Tuli A., and Sharma M.. 2017. The Rab7 effector PLEKHM1 binds Arl8b to promote cargo traffic to lysosomes . J. Cell Biol. 216 :1051–1070. 10.1083/jcb.201607085 [ Europe PMC free article ] [ Abstract ] [ CrossRef ] [ Google Scholar ]
- Matsui T., Jiang P., Nakano S., Sakamaki Y., Yamamoto H., and Mizushima N.. 2018. Autophagosomal YKT6 is required for fusion with lysosomes independently of syntaxin 17 . J. Cell Biol. 217 :2633–2645. 10.1083/jcb.201712058 [ Europe PMC free article ] [ Abstract ] [ CrossRef ] [ Google Scholar ]
- Matsunaga K., Saitoh T., Tabata K., Omori H., Satoh T., Kurotori N., Maejima I., Shirahama-Noda K., Ichimura T., Isobe T., et al. . 2009. Two Beclin 1-binding proteins, Atg14L and Rubicon, reciprocally regulate autophagy at different stages . Nat. Cell Biol. 11 :385–396. 10.1038/ncb1846 [ Abstract ] [ CrossRef ] [ Google Scholar ]
- Mauvezin C., Nagy P., Juhász G., and Neufeld T.P.. 2015. Autophagosome-lysosome fusion is independent of V-ATPase-mediated acidification . Nat. Commun. 6 :7007 10.1038/ncomms8007 [ Europe PMC free article ] [ Abstract ] [ CrossRef ] [ Google Scholar ]
- McEwan D.G., Popovic D., Gubas A., Terawaki S., Suzuki H., Stadel D., Coxon F.P., Miranda de Stegmann D., Bhogaraju S., Maddi K., et al. . 2015. PLEKHM1 regulates autophagosome-lysosome fusion through HOPS complex and LC3/GABARAP proteins . Mol. Cell. 57 :39–54. 10.1016/j.molcel.2014.11.006 [ Abstract ] [ CrossRef ] [ Google Scholar ]
- Medina D.L., Di Paola S., Peluso I., Armani A., De Stefani D., Venditti R., Montefusco S., Scotto-Rosato A., Prezioso C., Forrester A., et al. . 2015. Lysosomal calcium signalling regulates autophagy through calcineurin and TFEB . Nat. Cell Biol. 17 :288–299. 10.1038/ncb3114 [ Europe PMC free article ] [ Abstract ] [ CrossRef ] [ Google Scholar ]
- Miao G., Zhao Y.G., Zhao H., Ji C., Sun H., Chen Y., and Zhang H.. 2016. Mice deficient in the Vici syndrome gene Epg5 exhibit features of retinitis pigmentosa . Autophagy. 12 :2263–2270. 10.1080/15548627.2016.1238554 [ Europe PMC free article ] [ Abstract ] [ CrossRef ] [ Google Scholar ]
- Mizuno-Yamasaki E., Rivera-Molina F., and Novick P.. 2012. GTPase networks in membrane traffic . Annu. Rev. Biochem. 81 :637–659. 10.1146/annurev-biochem-052810-093700 [ Europe PMC free article ] [ Abstract ] [ CrossRef ] [ Google Scholar ]
- Nakatogawa H., Ichimura Y., and Ohsumi Y.. 2007. Atg8, a ubiquitin-like protein required for autophagosome formation, mediates membrane tethering and hemifusion . Cell. 130 :165–178. 10.1016/j.cell.2007.05.021 [ Abstract ] [ CrossRef ] [ Google Scholar ]
- Nakatogawa H., Suzuki K., Kamada Y., and Ohsumi Y.. 2009. Dynamics and diversity in autophagy mechanisms: lessons from yeast . Nat. Rev. Mol. Cell Biol. 10 :458–467. 10.1038/nrm2708 [ Abstract ] [ CrossRef ] [ Google Scholar ]
- Nguyen T.N., Padman B.S., Usher J., Oorschot V., Ramm G., and Lazarou M.. 2016. Atg8 family LC3/GABARAP proteins are crucial for autophagosome-lysosome fusion but not autophagosome formation during PINK1/Parkin mitophagy and starvation . J. Cell Biol. 215 :857–874. 10.1083/jcb.201607039 [ Europe PMC free article ] [ Abstract ] [ CrossRef ] [ Google Scholar ]
- Nixon R.A., Yang D.S., and Lee J.H.. 2008. Neurodegenerative lysosomal disorders: a continuum from development to late age . Autophagy. 4 :590–599. 10.4161/auto.6259 [ Abstract ] [ CrossRef ] [ Google Scholar ]
- Nordmann M., Cabrera M., Perz A., Bröcker C., Ostrowicz C., Engelbrecht-Vandré S., and Ungermann C.. 2010. The Mon1-Ccz1 complex is the GEF of the late endosomal Rab7 homolog Ypt7 . Curr. Biol. 20 :1654–1659. 10.1016/j.cub.2010.08.002 [ Abstract ] [ CrossRef ] [ Google Scholar ]
- Orsi A., Razi M., Dooley H.C., Robinson D., Weston A.E., Collinson L.M., and Tooze S.A.. 2012. Dynamic and transient interactions of Atg9 with autophagosomes, but not membrane integration, are required for autophagy . Mol. Biol. Cell. 23 :1860–1873. 10.1091/mbc.e11-09-0746 [ Europe PMC free article ] [ Abstract ] [ CrossRef ] [ Google Scholar ]
- Pankiv S., Alemu E.A., Brech A., Bruun J.A., Lamark T., Overvatn A., Bjørkøy G., and Johansen T.. 2010. FYCO1 is a Rab7 effector that binds to LC3 and PI3P to mediate microtubule plus end-directed vesicle transport . J. Cell Biol. 188 :253–269. 10.1083/jcb.200907015 [ Europe PMC free article ] [ Abstract ] [ CrossRef ] [ Google Scholar ]
- Parkinson N., Ince P.G., Smith M.O., Highley R., Skibinski G., Andersen P.M., Morrison K.E., Pall H.S., Hardiman O., Collinge J., et al. FReJA Consortium . 2006. ALS phenotypes with mutations in CHMP2B (charged multivesicular body protein 2B) . Neurology. 67 :1074–1077. 10.1212/01.wnl.0000231510.89311.8b [ Abstract ] [ CrossRef ] [ Google Scholar ]
- Peng C., Ye J., Yan S., Kong S., Shen Y., Li C., Li Q., Zheng Y., Deng K., Xu T., and Tao W.. 2012. Ablation of vacuole protein sorting 18 (Vps18) gene leads to neurodegeneration and impaired neuronal migration by disrupting multiple vesicle transport pathways to lysosomes . J. Biol. Chem. 287 :32861–32873. 10.1074/jbc.M112.384305 [ Europe PMC free article ] [ Abstract ] [ CrossRef ] [ Google Scholar ]
- Phillips M.J., and Voeltz G.K.. 2016. Structure and function of ER membrane contact sites with other organelles . Nat. Rev. Mol. Cell Biol. 17 :69–82. 10.1038/nrm.2015.8 [ Europe PMC free article ] [ Abstract ] [ CrossRef ] [ Google Scholar ]
- Pontano Vaites L., Paulo J.A., Huttlin E.L., and Harper J.W.. 2017. Systematic analysis of human cells lacking ATG8 proteins uncovers roles for GABARAPs and the CCZ1/MON1 regulator C18orf8/RMC1 in macro and selective autophagic flux . Mol. Cell. Biol. 38 :MCB.00392-17. [ Europe PMC free article ] [ Abstract ] [ Google Scholar ]
- Prinz W.A. 2014. Bridging the gap: membrane contact sites in signaling, metabolism, and organelle dynamics . J. Cell Biol. 205 :759–769. 10.1083/jcb.201401126 [ Europe PMC free article ] [ Abstract ] [ CrossRef ] [ Google Scholar ]
- Pu J., Guardia C.M., Keren-Kaplan T., and Bonifacino J.S.. 2016. Mechanisms and functions of lysosome positioning . J. Cell Sci. 129 :4329–4339. 10.1242/jcs.196287 [ Europe PMC free article ] [ Abstract ] [ CrossRef ] [ Google Scholar ]
- Puri C., Renna M., Bento C.F., Moreau K., and Rubinsztein D.C.. 2013. Diverse autophagosome membrane sources coalesce in recycling endosomes . Cell. 154 :1285–1299. 10.1016/j.cell.2013.08.044 [ Europe PMC free article ] [ Abstract ] [ CrossRef ] [ Google Scholar ]
- Raben N., and Puertollano R.. 2016. TFEB and TFE3: Linking Lysosomes to Cellular Adaptation to Stress . Annu. Rev. Cell Dev. Biol. 32 :255–278. 10.1146/annurev-cellbio-111315-125407 [ Europe PMC free article ] [ Abstract ] [ CrossRef ] [ Google Scholar ]
- Ravikumar B., Moreau K., Jahreiss L., Puri C., and Rubinsztein D.C.. 2010. Plasma membrane contributes to the formation of pre-autophagosomal structures . Nat. Cell Biol. 12 :747–757. 10.1038/ncb2078 [ Europe PMC free article ] [ Abstract ] [ CrossRef ] [ Google Scholar ]
- Razi M., Chan E.Y., and Tooze S.A.. 2009. Early endosomes and endosomal coatomer are required for autophagy . J. Cell Biol. 185 :305–321. 10.1083/jcb.200810098 [ Europe PMC free article ] [ Abstract ] [ CrossRef ] [ Google Scholar ]
- Rocha N., Kuijl C., van der Kant R., Janssen L., Houben D., Janssen H., Zwart W., and Neefjes J.. 2009. Cholesterol sensor ORP1L contacts the ER protein VAP to control Rab7-RILP-p150 Glued and late endosome positioning . J. Cell Biol. 185 :1209–1225. 10.1083/jcb.200811005 [ Europe PMC free article ] [ Abstract ] [ CrossRef ] [ Google Scholar ]
- Rusten T.E., Vaccari T., Lindmo K., Rodahl L.M., Nezis I.P., Sem-Jacobsen C., Wendler F., Vincent J.P., Brech A., Bilder D., and Stenmark H.. 2007. ESCRTs and Fab1 regulate distinct steps of autophagy . Curr. Biol. 17 :1817–1825. 10.1016/j.cub.2007.09.032 [ Abstract ] [ CrossRef ] [ Google Scholar ]
- Sato M., Saegusa K., Sato K., Hara T., Harada A., and Sato K.. 2011. Caenorhabditis elegans SNAP-29 is required for organellar integrity of the endomembrane system and general exocytosis in intestinal epithelial cells . Mol. Biol. Cell. 22 :2579–2587. 10.1091/mbc.e11-04-0279 [ Europe PMC free article ] [ Abstract ] [ CrossRef ] [ Google Scholar ]
- Settembre C., Di Malta C., Polito V.A., Garcia Arencibia M., Vetrini F., Erdin S., Erdin S.U., Huynh T., Medina D., Colella P., et al. . 2011. TFEB links autophagy to lysosomal biogenesis . Science. 332 :1429–1433. 10.1126/science.1204592 [ Europe PMC free article ] [ Abstract ] [ CrossRef ] [ Google Scholar ]
- Settembre C., Zoncu R., Medina D.L., Vetrini F., Erdin S., Erdin S., Huynh T., Ferron M., Karsenty G., Vellard M.C., et al. . 2012. A lysosome-to-nucleus signalling mechanism senses and regulates the lysosome via mTOR and TFEB . EMBO J. 31 :1095–1108. 10.1038/emboj.2012.32 [ Europe PMC free article ] [ Abstract ] [ CrossRef ] [ Google Scholar ]
- Skibinski G., Parkinson N.J., Brown J.M., Chakrabarti L., Lloyd S.L., Hummerich H., Nielsen J.E., Hodges J.R., Spillantini M.G., Thusgaard T., et al. . 2005. Mutations in the endosomal ESCRTIII-complex subunit CHMP2B in frontotemporal dementia . Nat. Genet. 37 :806–808. 10.1038/ng1609 [ Abstract ] [ CrossRef ] [ Google Scholar ]
- Slawson C., Copeland R.J., and Hart G.W.. 2010. O-GlcNAc signaling: a metabolic link between diabetes and cancer? Trends Biochem. Sci. 35 :547–555. 10.1016/j.tibs.2010.04.005 [ Europe PMC free article ] [ Abstract ] [ CrossRef ] [ Google Scholar ]
- Stenmark H. 2009. Rab GTPases as coordinators of vesicle traffic . Nat. Rev. Mol. Cell Biol. 10 :513–525. 10.1038/nrm2728 [ Abstract ] [ CrossRef ] [ Google Scholar ]
- Stolz A., Ernst A., and Dikic I.. 2014. Cargo recognition and trafficking in selective autophagy . Nat. Cell Biol. 16 :495–501. 10.1038/ncb2979 [ Abstract ] [ CrossRef ] [ Google Scholar ]
- Stroupe C., Collins K.M., Fratti R.A., and Wickner W.. 2006. Purification of active HOPS complex reveals its affinities for phosphoinositides and the SNARE Vam7p . EMBO J. 25 :1579–1589. 10.1038/sj.emboj.7601051 [ Europe PMC free article ] [ Abstract ] [ CrossRef ] [ Google Scholar ]
- Sun Q., Westphal W., Wong K.N., Tan I., and Zhong Q.. 2010. Rubicon controls endosome maturation as a Rab7 effector . Proc. Natl. Acad. Sci. USA. 107 :19338–19343. 10.1073/pnas.1010554107 [ Europe PMC free article ] [ Abstract ] [ CrossRef ] [ Google Scholar ]
- Szatmári Z., and Sass M.. 2014. The autophagic roles of Rab small GTPases and their upstream regulators: a review . Autophagy. 10 :1154–1166. 10.4161/auto.29395 [ Europe PMC free article ] [ Abstract ] [ CrossRef ] [ Google Scholar ]
- Tabata K., Matsunaga K., Sakane A., Sasaki T., Noda T., and Yoshimori T.. 2010. Rubicon and PLEKHM1 negatively regulate the endocytic/autophagic pathway via a novel Rab7-binding domain . Mol. Biol. Cell. 21 :4162–4172. 10.1091/mbc.e10-06-0495 [ Europe PMC free article ] [ Abstract ] [ CrossRef ] [ Google Scholar ]
- Takahashi Y., He H., Tang Z., Hattori T., Liu Y., Young M.M., Serfass J.M., Chen L., Gebru M., Chen C., et al. . 2018. An autophagy assay reveals the ESCRT-III component CHMP2A as a regulator of phagophore closure . Nat. Commun. 9 :2855 10.1038/s41467-018-05254-w [ Europe PMC free article ] [ Abstract ] [ CrossRef ] [ Google Scholar ]
- Takáts S., Nagy P., Varga Á., Pircs K., Kárpáti M., Varga K., Kovács A.L., Hegedűs K., and Juhász G.. 2013. Autophagosomal Syntaxin17-dependent lysosomal degradation maintains neuronal function in Drosophila . J. Cell Biol. 201 :531–539. 10.1083/jcb.201211160 [ Europe PMC free article ] [ Abstract ] [ CrossRef ] [ Google Scholar ]
- Takáts S., Pircs K., Nagy P., Varga Á., Kárpáti M., Hegedűs K., Kramer H., Kovács A.L., Sass M., and Juhász G.. 2014. Interaction of the HOPS complex with Syntaxin 17 mediates autophagosome clearance in Drosophila . Mol. Biol. Cell. 25 :1338–1354. 10.1091/mbc.e13-08-0449 [ Europe PMC free article ] [ Abstract ] [ CrossRef ] [ Google Scholar ]
- Tanaka Y., Guhde G., Suter A., Eskelinen E.L., Hartmann D., Lüllmann-Rauch R., Janssen P.M., Blanz J., von Figura K., and Saftig P.. 2000. Accumulation of autophagic vacuoles and cardiomyopathy in LAMP-2-deficient mice . Nature. 406 :902–906. 10.1038/35022595 [ Abstract ] [ CrossRef ] [ Google Scholar ]
- Tian Y., Li Z., Hu W., Ren H., Tian E., Zhao Y., Lu Q., Huang X., Yang P., Li X., et al. . 2010. C. elegans screen identifies autophagy genes specific to multicellular organisms . Cell. 141 :1042–1055. 10.1016/j.cell.2010.04.034 [ Abstract ] [ CrossRef ] [ Google Scholar ]
- Tooze J., Hollinshead M., Ludwig T., Howell K., Hoflack B., and Kern H.. 1990. In exocrine pancreas, the basolateral endocytic pathway converges with the autophagic pathway immediately after the early endosome . J. Cell Biol. 111 :329–345. 10.1083/jcb.111.2.329 [ Europe PMC free article ] [ Abstract ] [ CrossRef ] [ Google Scholar ]
- Tsuboyama K., Koyama-Honda I., Sakamaki Y., Koike M., Morishita H., and Mizushima N.. 2016. The ATG conjugation systems are important for degradation of the inner autophagosomal membrane . Science. 354 :1036–1041. 10.1126/science.aaf6136 [ Abstract ] [ CrossRef ] [ Google Scholar ]
- Urwin H., Authier A., Nielsen J.E., Metcalf D., Powell C., Froud K., Malcolm D.S., Holm I., Johannsen P., Brown J., et al. FReJA Consortium . 2010. Disruption of endocytic trafficking in frontotemporal dementia with CHMP2B mutations . Hum. Mol. Genet. 19 :2228–2238. 10.1093/hmg/ddq100 [ Europe PMC free article ] [ Abstract ] [ CrossRef ] [ Google Scholar ]
- Wang H., Sun H.Q., Zhu X., Zhang L., Albanesi J., Levine B., and Yin H.. 2015. GABARAPs regulate PI4P-dependent autophagosome:lysosome fusion . Proc. Natl. Acad. Sci. USA. 112 :7015–7020. 10.1073/pnas.1507263112 [ Europe PMC free article ] [ Abstract ] [ CrossRef ] [ Google Scholar ]
- Wang Z., Miao G., Xue X., Guo X., Yuan C., Wang Z., Zhang G., Chen Y., Feng D., Hu J., and Zhang H.. 2016. The Vici Syndrome Protein EPG5 Is a Rab7 Effector that Determines the Fusion Specificity of Autophagosomes with Late Endosomes/Lysosomes . Mol. Cell. 63 :781–795. 10.1016/j.molcel.2016.08.021 [ Abstract ] [ CrossRef ] [ Google Scholar ]
- Weidberg H., Shvets E., Shpilka T., Shimron F., Shinder V., and Elazar Z.. 2010. LC3 and GATE-16/GABARAP subfamilies are both essential yet act differently in autophagosome biogenesis . EMBO J. 29 :1792–1802. 10.1038/emboj.2010.74 [ Europe PMC free article ] [ Abstract ] [ CrossRef ] [ Google Scholar ]
- Weidberg H., Shpilka T., Shvets E., Abada A., Shimron F., and Elazar Z.. 2011. LC3 and GATE-16 N termini mediate membrane fusion processes required for autophagosome biogenesis . Dev. Cell. 20 :444–454. 10.1016/j.devcel.2011.02.006 [ Abstract ] [ CrossRef ] [ Google Scholar ]
- Wijdeven R.H., Janssen H., Nahidiazar L., Janssen L., Jalink K., Berlin I., and Neefjes J.. 2016. Cholesterol and ORP1L-mediated ER contact sites control autophagosome transport and fusion with the endocytic pathway . Nat. Commun. 7 :11808 10.1038/ncomms11808 [ Europe PMC free article ] [ Abstract ] [ CrossRef ] [ Google Scholar ]
- Wu F., Watanabe Y., Guo X.Y., Qi X., Wang P., Zhao H.Y., Wang Z., Fujioka Y., Zhang H., Ren J.Q., et al. . 2015. Structural Basis of the Differential Function of the Two C. elegans Atg8 Homologs, LGG-1 and LGG-2, in Autophagy . Mol. Cell. 60 :914–929. 10.1016/j.molcel.2015.11.019 [ Abstract ] [ CrossRef ] [ Google Scholar ]
- Wu Y., Cheng S., Zhao H., Zou W., Yoshina S., Mitani S., Zhang H., and Wang X.. 2014. PI3P phosphatase activity is required for autophagosome maturation and autolysosome formation . EMBO Rep. 15 :973–981. 10.15252/embr.201438618 [ Europe PMC free article ] [ Abstract ] [ CrossRef ] [ Google Scholar ]
- Ylä-Anttila P., Vihinen H., Jokitalo E., and Eskelinen E.L.. 2009. 3D tomography reveals connections between the phagophore and endoplasmic reticulum . Autophagy. 5 :1180–1185. 10.4161/auto.5.8.10274 [ Abstract ] [ CrossRef ] [ Google Scholar ]
- Yokota S., Himeno M., and Kato K.. 1995. Formation of autophagosomes during degradation of excess peroxisomes induced by di-(2-ethylhexyl)-phthalate treatment. III. Fusion of early autophagosomes with lysosomal compartments . Eur. J. Cell Biol. 66 :15–24. [ Abstract ] [ Google Scholar ]
- Yu I.M., and Hughson F.M.. 2010. Tethering factors as organizers of intracellular vesicular traffic . Annu. Rev. Cell Dev. Biol. 26 :137–156. 10.1146/annurev.cellbio.042308.113327 [ Abstract ] [ CrossRef ] [ Google Scholar ]
- Yu S., and Melia T.J.. 2017. The coordination of membrane fission and fusion at the end of autophagosome maturation . Curr. Opin. Cell Biol. 47 :92–98. 10.1016/j.ceb.2017.03.010 [ Europe PMC free article ] [ Abstract ] [ CrossRef ] [ Google Scholar ]
- Zhang H., and Baehrecke E.H.. 2015. Eaten alive: novel insights into autophagy from multicellular model systems . Trends Cell Biol. 25 :376–387. 10.1016/j.tcb.2015.03.001 [ Europe PMC free article ] [ Abstract ] [ CrossRef ] [ Google Scholar ]
- Zhang H., and Hu J.J.. 2016. Shaping the endoplasmic reticulum into a social network . Trends Cell Biol. 26 :934–943. 10.1016/j.tcb.2016.06.002 [ Abstract ] [ CrossRef ] [ Google Scholar ]
- Zhang X., Wang L., Lak B., Li J., Jokitalo E., and Wang Y.. 2018. GRASP55 Senses Glucose Deprivation through O-GlcNAcylation to Promote Autophagosome-Lysosome Fusion . Dev. Cell. 45 :245–261.e6. 10.1016/j.devcel.2018.03.023 [ Abstract ] [ CrossRef ] [ Google Scholar ]
- Zhao Y.G., and Zhang H.. 2018. Formation and maturation of autophagosomes in higher eukaryotes: a social network . Curr. Opin. Cell Biol. 53 :29–36. 10.1016/j.ceb.2018.04.003 [ Abstract ] [ CrossRef ] [ Google Scholar ]
- Zhao H., Zhao Y.G., Wang X., Xu L., Miao L., Feng D., Chen Q., Kovács A.L., Fan D., and Zhang H.. 2013a Mice deficient in Epg5 exhibit selective neuronal vulnerability to degeneration . J. Cell Biol. 200 :731–741. 10.1083/jcb.201211014 [ Europe PMC free article ] [ Abstract ] [ CrossRef ] [ Google Scholar ]
- Zhao Y.G., Zhao H., Miao L., Wang L., Sun F., and Zhang H.. 2012. The p53-induced gene Ei24 is an essential component of the basal autophagy pathway . J. Biol. Chem. 287 :42053–42063. 10.1074/jbc.M112.415968 [ Europe PMC free article ] [ Abstract ] [ CrossRef ] [ Google Scholar ]
- Zhao Y.G., Zhao H., Sun H., and Zhang H.. 2013b Role of Epg5 in selective neurodegeneration and Vici syndrome . Autophagy. 9 :1258–1262. 10.4161/auto.24856 [ Europe PMC free article ] [ Abstract ] [ CrossRef ] [ Google Scholar ]
- Zhao Y.G., Chen Y., Miao G., Zhao H., Qu W., Li D., Wang Z., Liu N., Li L., Chen S., et al. . 2017. The ER-Localized Transmembrane Protein EPG-3/VMP1 Regulates SERCA Activity to Control ER-Isolation Membrane Contacts for Autophagosome Formation . Mol. Cell. 67 :974–989.e6. 10.1016/j.molcel.2017.08.005 [ Abstract ] [ CrossRef ] [ Google Scholar ]
- Zhao Y.G., Liu N., Miao G., Chen Y., Zhao H., and Zhang H.. 2018. The ER Contact Proteins VAPA/B Interact with Multiple Autophagy Proteins to Modulate Autophagosome Biogenesis . Curr. Biol. 28 :1234–1245.e4. 10.1016/j.cub.2018.03.002 [ Abstract ] [ CrossRef ] [ Google Scholar ]
- Zhen Y., and Li W.. 2015. Impairment of autophagosome-lysosome fusion in the buff mutant mice with the VPS33A(D251E) mutation . Autophagy. 11 :1608–1622. 10.1080/15548627.2015.1072669 [ Europe PMC free article ] [ Abstract ] [ CrossRef ] [ Google Scholar ]
- Zhong Y., Wang Q.J., Li X., Yan Y., Backer J.M., Chait B.T., Heintz N., and Yue Z.. 2009. Distinct regulation of autophagic activity by Atg14L and Rubicon associated with Beclin 1-phosphatidylinositol-3-kinase complex . Nat. Cell Biol. 11 :468–476. 10.1038/ncb1854 [ Europe PMC free article ] [ Abstract ] [ CrossRef ] [ Google Scholar ]
Full text links
Read article at publisher's site: https://doi.org/10.1083/jcb.201810099
Citations & impact
Impact metrics, citations of article over time, alternative metrics.

Article citations
The interplay between autophagy and cgas-sting signaling and its implications for cancer..
Schmid M , Fischer P , Engl M , Widder J , Kerschbaum-Gruber S , Slade D
Front Immunol , 15:1356369, 10 Apr 2024
Cited by: 0 articles | PMID: 38660307 | PMCID: PMC11039819
Advancements in research on the immune-inflammatory mechanisms mediated by NLRP3 inflammasome in ischemic stroke and the regulatory role of natural plant products.
Yang K , Zeng L , He Q , Wang S , Xu H , Ge J
Front Pharmacol , 15:1250918, 27 Mar 2024
Cited by: 0 articles | PMID: 38601463 | PMCID: PMC11004298
MoMkk1 and MoAtg1 dichotomously regulating autophagy and pathogenicity through MoAtg9 phosphorylation in Magnaporthe oryzae .
Kong Y , Guo P , Xu J , Li J , Wu M , Zhang Z , Wang Y , Liu X , Yang L , Liu M , Zhang H , Wang P , Zhang Z
mBio , 15(4):e0334423, 19 Mar 2024
Cited by: 0 articles | PMID: 38501872 | PMCID: PMC11005334
Interplay between group A Streptococcus and host innate immune responses.
Su MS-W , Cheng Y-L , Lin Y-S , Wu J-J
Microbiol Mol Biol Rev , 88(1):e0005222, 07 Mar 2024
Cited by: 1 article | PMID: 38451081 | PMCID: PMC10966951
Review Free full text in Europe PMC
Recent progresses in the late stages of autophagy.
Zhu Y , Liu F , Jian F , Rong Y
Cell Insight , 3(2):100152, 08 Feb 2024
Cited by: 0 articles | PMID: 38435435 | PMCID: PMC10904915
Similar Articles
To arrive at the top five similar articles we use a word-weighted algorithm to compare words from the Title and Abstract of each citation.
Machinery, regulation and pathophysiological implications of autophagosome maturation.
Zhao YG , Codogno P , Zhang H
Nat Rev Mol Cell Biol , 22(11):733-750, 23 Jul 2021
Cited by: 143 articles | PMID: 34302147 | PMCID: PMC8300085
Membrane Trafficking in Autophagy.
Søreng K , Neufeld TP , Simonsen A
Int Rev Cell Mol Biol , 336:1-92, 21 Sep 2017
Cited by: 46 articles | PMID: 29413888
Quantitative 3D correlative light and electron microscopy of organelle association during autophagy.
Takahashi S , Saito C , Koyama-Honda I , Mizushima N
Cell Struct Funct , 47(2):89-99, 22 Nov 2022
Cited by: 6 articles | PMID: 36418108 | PMCID: PMC10511054
Coordination of Autophagosome-Lysosome Fusion by Atg8 Family Members.
Kriegenburg F , Ungermann C , Reggiori F
Curr Biol , 28(8):R512-R518, 01 Apr 2018
Cited by: 47 articles | PMID: 29689234
Formation and maturation of autophagosomes in higher eukaryotes: a social network.
Zhao YG , Zhang H
Curr Opin Cell Biol , 53:29-36, 01 May 2018
Cited by: 30 articles | PMID: 29727743
Funding
Funders who supported this work.
Chinese Academy of Sciences (2)
Grant ID: QYZDY-SSW-SMC006
4 publication s
Grant ID: XDB19000000
73 publication s
National Natural Science Foundation of China (3)
Grant ID: 31421002
13 publication s
Grant ID: 31561143001
7 publication s
Grant ID: 31630048
9 publication s
Orphan Disease Center (1)
Grant ID: MDBR-18-104-BPAN
1 publication
Europe PMC is part of the ELIXIR infrastructure

COMMENTS
Autophagosome maturation: An epic journey from the ER to lysosomes J Cell Biol. 2019 Mar 4;218(3):757-770. doi: 10.1083/jcb.201810099. Epub 2018 Dec 21. Authors ... Dysfunction of autophagosome maturation is associated with various human diseases, including neurodegenerative diseases, Vici syndrome, cancer, and lysosomal storage disorders. ...
The machinery mediating autophagosome maturation is under spatiotemporal control and provides regulatory nodes to integrate nutrient availability with autophagy activity. Dysfunction of autophagosome maturation is associated with various human diseases, including neurodegenerative diseases, Vici syndrome, cancer, and lysosomal storage disorders.
Autophagosome maturation: An epic journey from the ER to lysosomes Yan G. Zhao1 and Hong Zhang2,3 Introduction Macroautophagy (hereafter autophagy) refers to the engulfment of cytoplasmic contents, such as a portion of cytosol, mitochon-dria, or the ER, in a double-membrane autophagosome and their
Autophagosome maturation: An epic journey from the ER to lysosomes. December 2018; Journal of Cell Biology (JCB) 218(3): ... the ER t o lysosome s. Yan G. Zhao 1 and Hong Zhang 2,3 ...
Dysfunction of autophagosome maturation is associated with various human diseases, including neurodegenerative diseases, Vici syndrome, cancer, and lysosomal storage disorders, and understanding the molecular mechanisms underlying autophagy will provide new insights into the pathogenesis and treatment of these diseases. Macroautophagy involves the sequestration of cytoplasmic contents in a ...
Figure 3. Lysosome positioning modulates autophagosome-lysosome fusion efficiency. Center: Amphisome- and lysosome-localized ORP1L senses cholesterol levels. Under adequate cholesterol conditions, ORP1L binds to cholesterol on the membrane through its ORD domain, which further binds to Rab7/ RILP for subsequent recruitment of PLE KHM1 and HOPS to facilitate autophagosome-endolysosome ...
Macroautophagy involves the sequestration of cytoplasmic contents in a double-membrane autophagosome and their delivery to lysosomes for degradation. In multicellular organisms, nascent autophagosomes fuse with vesicles originating from endolysosomal compartments before forming degradative autolysosomes, a process known as autophagosome maturation. ATG8 family members, tethering factors, Rab ...
Following its biogenesis, the autophagosome fuses with the lysosome or vacuole to achieve degradation of the contents. In yeast, the autophagosomes are formed at the endoplasmic reticulum (ER ...
Zhao, Y. G. & Zhang, H. Autophagosome maturation: an epic journey from the ER to lysosomes. J. ... and also promotes autophagosome-lysosome fusion by recruiting the HOPS complex. ...
Introduction. Macroautophagy (hereafter autophagy) refers to the engulfment of cytoplasmic contents, such as a portion of cytosol, mitochondria, or the ER, in a double-membrane autophagosome and their delivery to the vacuole (in yeast) or lysosomes (in multicellular organisms) for degradation. Formation of the autophagosome involves multiple ...
Macroautophagy involves the sequestration of cytoplasmic contents in a double-membrane autophagosome and their delivery to lysosomes for degradation. In... Article OPEN ACCESS. Autophagosome maturation: An epic journey from the ER to lysosomes ... & Zhang, H. (2019, March 1). Autophagosome maturation: An epic journey from the ER to lysosomes ...
Macroautophagy (also known as autophagy) plays a pivotal role in maintaining cellular homeostasis. The terminal step of the multi-step autophagy degradation pathway involves fusion between the cargo-laden, double-membraned autophagosome and the lytic organelle lysosome/vacuole. Over the past decade, various core components of the molecular ...
Key Points. Autophagy is an evolutionarily conserved lysosome-mediated degradation process that involves membrane-bound organelles called autophagosomes. Macroautophagy, commonly referred to as ...
The fusion of autophagosome with lysosome is required for autophagic flux. EPG5, a RAB7A effector, ... Zhao YG, Zhang H. Autophagosome maturation: an epic journey from the ER to lysosomes. J Cell Biol. 2019; 218:757-770. doi: 10.1083/jcb.201810099. [PMC free article] ...
Macroautophagy involves the sequestration of cytoplasmic contents in a double-membrane autophagosome and their delivery to lysosomes for degradation. In multicellular organisms, nascent autophagosomes fuse with vesicles originating from endolysosomal compartments before forming degradative autolysosomes, a process known as autophagosome maturation.
Specific function in autophagosome maturation and lysosome fusion unknown ... An epic journey from the ER to lysosomes. J Cell Biol 218, 757-770. 10.1083/jcb.201810099. [PMC free article] [Google Scholar] 20. Choi A.M., Ryter S.W., and Levine B. (2013). Autophagy in human health and disease. ...
Macroautophagy (hereafter autophagy) refers to the engulfment of cytoplasmic contents, such as a portion of cytosol, mitochon- dria, or the ER, in a double-membrane autophagosome and their ...
Supporting: 2, Contrasting: 1, Mentioning: 185 - Macroautophagy involves the sequestration of cytoplasmic contents in a double-membrane autophagosome and their delivery to lysosomes for degradation. In multicellular organisms, nascent autophagosomes fuse with vesicles originating from endolysosomal compartments before forming degradative autolysosomes, a process known as autophagosome maturation.
In this issue of Developmental Cell, Miao et al. discover that the SARS-CoV-2 protein ORF3a inhibits autophagosome-lysosome fusion by dysregulating the HOPS complex. ... Autophagosome maturation: an epic journey from the ER to lysosomes. J. Cell Biol. 2019; 218: 757-770. ... Autophagosome maturation: an epic journey from the ER to lysosomes. J ...
This is followed by fusion of autophagosome with lysosome and degradation/recycling of sequestered cellular components to ... ATG9 binds ATG2 and WIPI proteins (ATG18 in yeast, the PI3P effectors), to participate in the early stage of autophagosome biogenesis from ER ... Zhang H. Autophagosome maturation: an epic journey from the ER to ...
Introduction. Macroautophagy (hereafter autophagy) refers to the engulfment of cytoplasmic contents, such as a portion of cytosol, mitochondria, or the ER, in a double-membrane autophagosome and their delivery to the vacuole (in yeast) or lysosomes (in multicellular organisms) for degradation. Formation of the autophagosome involves multiple ...
Ji et al. demonstrate that the β-propeller proteins WDR45 and WDR45B modulate autophagosome-lysosome fusion in neural cells. WDR45/45B interact with the tether protein EPG5 for its targeting to late endosomes/lysosomes and therefore promote the formation of SNARE-tether fusion complexes during autophagosome maturation into autolysosomes.
Conversely, when a decrease in autophagic flux is suggested, the efficiency of autophagosome-lysosome fusion or lysosomal function should be checked, in addition to the autophagy-inducing signals. ... Autophagosome maturation: an epic journey from the ER to lysosomes. J. Cell Biol. 2018; 218: 757-770. Crossref;